Assessment of the Relevance of the Inclusion of Radionuclides as a Chemical of Mutual Concern under Annex 3 of the Canada-United States Great Lakes Water Quality Agreement
Executive Summary
The Great Lakes Water Quality Agreement (GLWQA) is an agreement between Canada and the United States of America (U.S.), first signed in 1972. It was amended in 2012, and includes Annex 3: Chemicals of Mutual Concern (CMCs). Through the annex, Canada and the U.S. have committed to:
“…contribute to the achievement of the General and Specific Objectives of this Agreement by protecting human health and the environment through cooperative and coordinated measures to reduce the anthropogenic release of chemicals of mutual concern into the Waters of the Great Lakes.”
Annex 3 commits the parties to identify and designate, on an ongoing basis, CMCs in the Great Lakes, which originate from anthropogenic sources and that both parties may be harmful to the environment or human health.
Under Annex 3, it is the responsibility of the parties to nominate substances for review and evaluation for potential designation as CMCs. As a means to foster enhanced GLWQA stakeholder engagement, the Great Lakes Executive Committee co-chairs agreed to introduce a process by which stakeholders in Canada and U.S. may formally propose the designation of specific chemicals. Following this process, the Canadian Environmental Law Association nominated radionuclides for consideration as candidate substances for classification as CMCs. A supporting letter was also submitted by 110 environmental, health and other advocacy groups.
This report evaluates the request for the designation of radionuclides as a candidate CMC under Annex 3. The evaluation was completed by the Canadian Nuclear Safety Commission (CNSC), at the request of Environment and Climate Change Canada. The CNSC is the federal agency responsible for regulating the use of nuclear energy and materials to protect health, safety, security and the environment; to implement Canada's international commitments on the peaceful use of nuclear energy; and to disseminate objective scientific, technical and regulatory information to the public.
Part I of this report provides a review of the current state of the science with respect to the health and environmental risks associated with radionuclides. The environmental and human health consequences of the radionuclides measured in the Great Lakes were evaluated and assessed against internationally and nationally recognized environmental and human health benchmarks. Based on an assessment using the best available science, there is no evidence to indicate that radionuclides currently within the Great Lakes are posing an unreasonable risk to the environment, or human health and safety.
Part II of this report presents the existing international and national risk management activities with respect to legislation and regulation on the development, production and use of nuclear energy, and the possession and use of nuclear substances (i.e., radionuclides). The transparency of these regulatory processes and the opportunities for public participation in the regulatory process are discussed. Governmental risk-management programs, monitoring programs and research activities are also presented.
Radionuclides are currently among the most heavily regulated substances in the world. Canada has an independent national nuclear regulatory body (i.e., the CNSC), the mandate of which is to ensure Canada's nuclear industry is protective of the environment and the health and safety of persons. Strong intergovernmental relationships have been established both federally and provincially to ensure radionuclides and radiation are safely managed.
The report concludes that radionuclides are not recommended as a candidate CMC for further evaluation under Annex 3. However, it identifies opportunities to improve the public availability of, and access to, release and monitoring data associated with the nuclear fuel cycle in Canada, and the need to continue to improve coordination and collaboration among various stakeholders on science priorities, research, surveillance and monitoring activities in the Great Lakes basin ecosystem
Table of contents
- 1.0 INTRODUCTION
- 2.0 IONIZING RADIATION AND HUMAN HEALTH
- 3.0 RADIONUCLIDES IN THE GREAT LAKES
- 4.0 GENERIC RISK ASSESSMENT
- 5.0 INTERNATIONAL AND NATIONAL SCIENCE AND REGULATORY FRAMEWORKS
- 6.0 CONCLUSIONS AND RECOMMENDATIONS
- ACRONYMS
- APPENDIX A: CNSC RADIONUCLIDE SINGLE MEDIA SCREENING CRITERION – FISH CONSUMPTION
- APPENDIX B: HUMAN HEALTH RISK ASSESSMENT: PUBLIC DOSE FROM GREAT LAKES EXPOSURE PATHWAYS
- APPENDIX C: RADIOLOGICAL ECOLOGICAL RISK ASSESSMENT
Introduction
The Great Lakes Water Quality Agreement (GLWQA) is an agreement between Canada and the United States of America (U.S.), first signed in 1972. It was amended in 2012, and includes Annex 3: Chemicals of Mutual Concern (CMCs). Through the Annex, Canada and the U.S. have committed to:
“…contribute to the achievement of the General and Specific Objectives of this Agreement by protecting human health and the environment through cooperative and coordinated measures to reduce the anthropogenic release of chemicals of mutual concern into the Waters of the Great Lakes.”
Annex 3 commits the parties to identify and designate, on an ongoing basis, CMCs in the Great Lakes, which originate
from anthropogenic sources and that are agreed to by both parties as being potentially harmful to the environment or
human health.
Under Annex 3, it is the responsibility of the parties to nominate substances for review and evaluation for
potential designation as CMCs. As a means to foster enhanced GLWQA stakeholder engagement, the Great Lakes Executive
Committee co-chairs agreed to introduce a process by which stakeholders in Canada and U.S. may formally propose
specific chemicals.
Proposals must be accompanied by supporting rationale that outlines the justification for the proposal. This supporting rationale should be based on currently available data or other information.
Radionuclides were nominated as a candidate CMC in March 2016 by the Canadian Environmental Law Association (CELA) and supported by 110 environmental, health and advocacy groups across the U.S. and Canada, including the Great Lakes Indian Fish & Wildlife Commission, which represents 11 tribal governments in the U.S.
This report evaluates the nomination of radionuclides as a candidate CMC under Annex 3. The evaluation was completed by the Canadian Nuclear Safety Commission (CNSC) at the request of Environment and Climate Change Canada. The CNSC is the federal agency responsible for regulating the use of nuclear energy and materials to protect health, safety, security and the environment; to implement Canada's international commitments on the peaceful use of nuclear energy; and to disseminate objective scientific, technical and regulatory information to the public.
Part I of this report provides a review of the current state of the science with respect to health and environmental risks associated with radionuclides. The quantities of radionuclides released to and measurable within the Great Lakes are evaluated and assessed against internationally and nationally recognized environmental and human health benchmarks.
Part II of this report presents the existing international and national activities with respect to legislation and regulation of the development, production and use of nuclear energy and the possession and use of nuclear substances (i.e., radionuclides). The transparency of these regulatory processes and the opportunities for public participation in the regulatory process are discussed. Governmental risk management and monitoring programs and research activities are also presented. Radionuclides are currently among the most heavily regulated substances in the world. Canada has an independent national nuclear regulatory body (i.e., the CNSC) with a mandate to protect the environment, health and safety with no promotional role for the industry. Strong intergovernmental relationships have been established both federally and provincially to ensure radionuclides and radiation are safely managed
Throughout the main report, reference is made to the accompanying appendices where more detailed supportive technical information is provided.
Part I: Science and assessment
2.0 Ionizing radiation and human health
2.1 Basics of radiation science
This section describes the basic science of radiation that is needed to understand the discussions in the remainder of this report, including the units for releases of nuclear substances to the environment, exposures of people and the environment to radiation, and the health implications of these exposures.
Radioactive materials (also called radionuclides or radioisotopes) consist of atoms, the nuclei of which have unstable ratios of neutrons and protons. Where a nucleus is unstable and has excess energy, the energy will be discharged during a decay process. Radioactive decay occurs when a radioactive atom releases its excess energy and is transformed to a more stable state, which may or may not be radioactive. This excess energy is carried by one or more subatomic particles and/or one or more gamma ray photons. These subatomic particles and photons collectively are referred to as radiation. Such radiation has enough energy to remove an electron from an atom, leaving it electrically charged – or ionized. Consequently, radiation emitted by radionuclides is classified as ionizing radiation. Non-ionizing radiation includes microwaves, infrared and ultraviolet radiation. It does not possess enough energy to ionize atoms and thus is not considered in this report.
The rate at which a sample of radionuclides decays, as described above, is called the activity of that sample. The International System of Units (SI unit) for activity is the becquerel (Bq). One becquerel of activity is equal to one nuclear transformation per second. The time it takes for a radioisotope to decay to half its starting activity is called the radiological half-life.
Radionuclide specific half-lives vary immensely – from fractions of a second to billions of years. Stable elements do not have half-lives. Thus, a given quantity of a chemically toxic element such as arsenic remains toxic forever, while a radionuclide loses its radiotoxicity through decay processes, and becomes a stable element over time.
As stated above, through the process of radioactive decay, one radioactive element is transformed to another in a more stable state. This new radionuclide or decay product is the daughter or progeny of the previous one (i.e., the parent). Where the resultant progeny is itself unstable, decay continues until a stable nuclide is attained. Each radionuclide generated along this chain has unique physical, chemical and radiological characteristics of half-life and type of radiation emitted as it decays. An example of a relatively complex but more commonly recognized decay chain is that of uranium-238. This chain is shown in figure 1 along with the basic types of radioactive decay associated with each step in the chain.
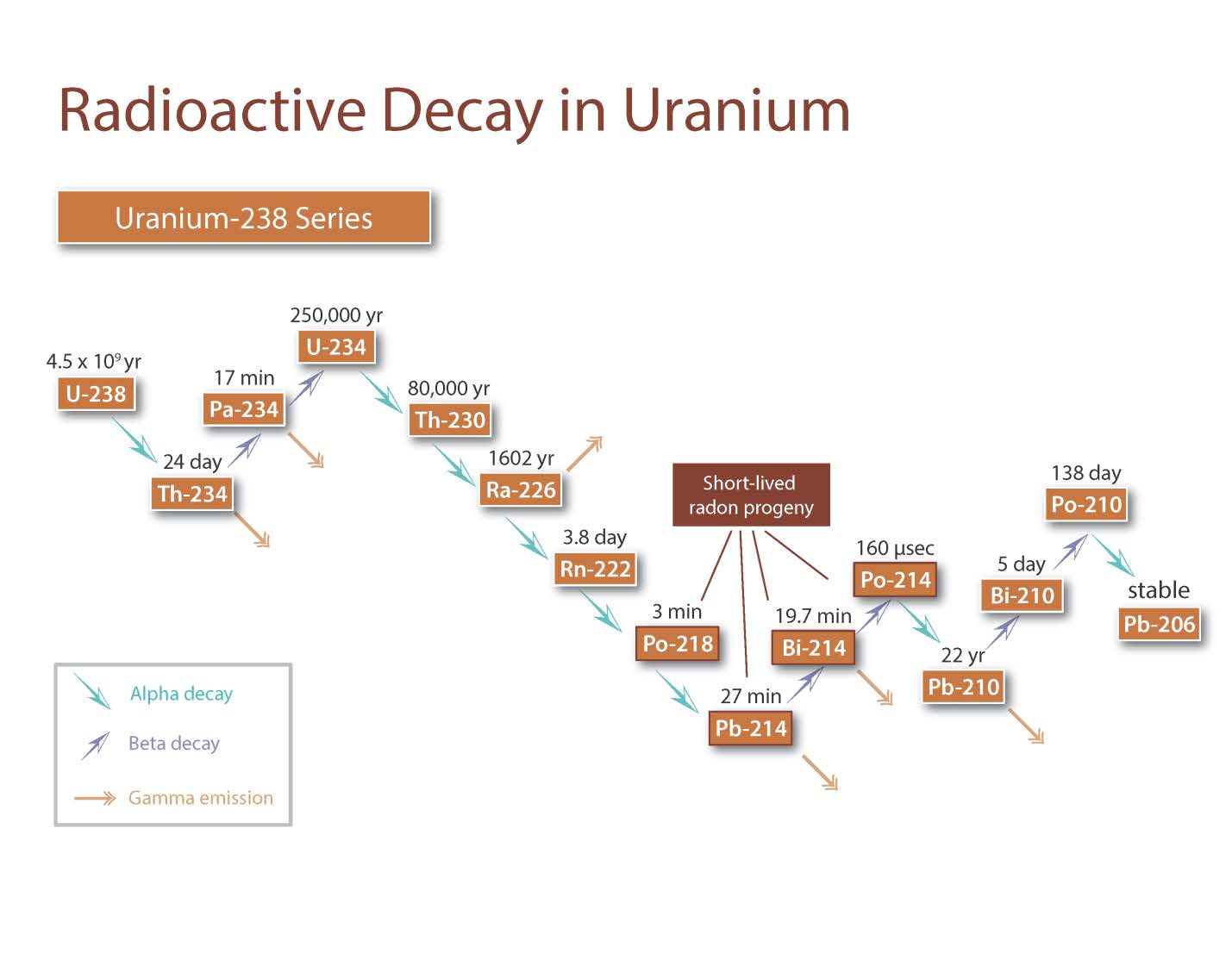
Descriptive text for Figure 1
Figure 1 is a visualization of the uranium 238 decay series to showcase that a relatively common radionuclide can have a complex decay series with multiple decays emitting alpha, beta, and gamma radiation. Uranium-238 decay chain consists of 13 unstable progeny ultimately ending with stable lead. The half-lives for this chain range from billions of years to fractions of a second.
In general, radioactive decay can be simplified into three basic forms:
- alpha radiation
- beta radiation
- gamma radiation
Alpha radiation involves the release of alpha particles which are identical to the nuclei of helium atoms, being a cluster of two neutrons and two protons (4He+2). These are relatively large, charged (+2), but slow-moving particles with limited ability to penetrate matter. For example, an alpha particle can move through only a few centimetres of air and can be blocked by a sheet of paper. Beta radiation involves the transformation of a neutron within the nucleus into a proton with the associated emission of a charged energetic electron called a beta particle. Beta particles are generally faster than alpha particles. They are capable of travelling through a few metres of air and can be blocked by a sheet of aluminum or a few millimeters of glass.
Gamma radiation consists of electromagnetic waves similar to X-rays of no charge or mass. Gamma radiation has significant penetrating power. It can move great distances through air and requires high-density materials, such as lead, to block its passage.
These types of radiation and their characteristic interactions with matter, more specifically biological tissue, will be discussed in more detail when addressing the health risks associated with radionuclides.
2.2 Calculation of dose
When alpha or beta particles or gamma photons penetrating matter pass close to an atom, there is the potential for interaction with the atom's electrons. While the exact process differs for each type of radiation, this interaction can result in the ionization of the atom.
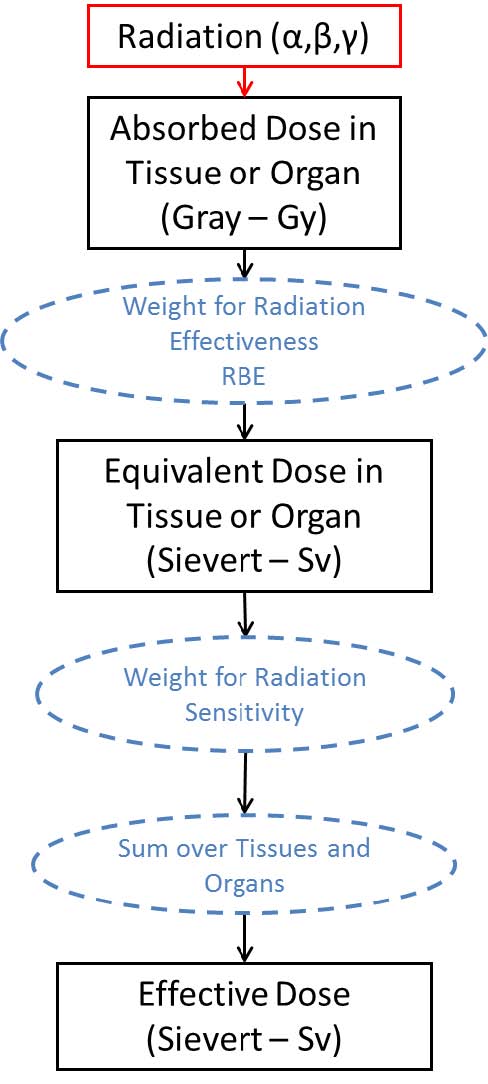
Descriptive text for Figure 2
Figure 2 demonstrates the hierarchical approach to how radiation effects on tissue are determined. When ionizing radiation passes through tissue or an organ, energy is deposited in that tissue, referred to the absorbed dose, measured in Grays. The absorbed dose is then modified by a weighting factor for radiation effectiveness (i.e., damage) or RBE which differs for alpha, beta and gamma radiation to determine the equivalent dose to that tissue or organ. This equivalent dose is measured in units known as Sieverts. The equivalent dose can then be further refined by accounting for radiation sensitivity of specific organs or tissues. Summing this dose across all the organs or tissues provides the effective dose, also measured in Sieverts.
Alpha particles with their high charges and mass create concentrated ionization. In the process, they lose energy – and thus their ability to penetrate a material – rapidly. Beta particles with their lower charges and mass cause less concentrated ionization. This, combined with their high speed, enables them to penetrate further through materials, although their path is less linear due to deflections arising from each interaction.
Since gamma photons have no mass or charge, they behave quite differently. They can pass near electrons with no effect until by chance they collide with electrons, atoms or nuclei. Collisions can result in the release of energetic charged particles, such as electrons. These charged particles in turn can result in ionization. Thus gamma photons can penetrate deeply due to the low concentration of ionizations produced as they travel through a material.
Dose: Absorbed ⇒ Equivalent ⇒ Effective
When radiation penetrates matter such as biological tissues, energy is deposited. The amount of energy deposited or absorbed from exposure to radiation is called a dose. It is necessary to understand the basic concepts of dose calculations if the potential health effects of radionuclides are to be understood. Radiation dose calculations are based on a hierarchical approach that begins with the absorbed dose, moves to an equivalent dose and finishes with an effective dose as shown in figure 2 and discussed below.
When ionizing radiation passes through tissue, the structure of molecules may be changed if the atoms these molecules contain are ionized. If the molecule is a part of the cell structure, the cell may be damaged. If the molecule is a component of the genetic material of the cell (e.g., DNA), then the behaviour or functioning of the cell may be altered.
The effect on a tissue is considered to be proportional to the energy deposited. The amount of radiation energy (measured in joules) absorbed per kilogram of a material is the absorbed dose. Its SI unit is the gray (Gy). Equal absorbed doses do not necessarily have the same biological effects. For example, an absorbed dose of 0.1 Gy of alpha radiation is about 20 times more harmful than the same absorbed dose provided by beta or gamma radiation.
This is considered by weighting the absorbed dose with a factor related to the type of radiation (i.e., alpha, beta or gamma). To calculate the dose to a tissue or organ, the absorbed dose is multiplied by the relevant weighting factor (wr) to obtain the equivalent dose, which is reported in sieverts (Sv). The overall equivalent dose to a tissue or organ (HT) is provided by the following equation:
where DT,R is the absorbed dose averaged over the tissue (T) due to radiation (R) and wr is the radiation weighting factor.
An equivalent dose of 1 Sv due to beta particles is expected to result in an equivalent biological effect as an equivalent dose of 1 Sv due to alpha particles. However, tissues themselves vary with respect to their radiosensitivity for cancer induction. For example, gonads are more sensitive than the thyroid. These differences in the various tissues' susceptibility to cancer induction must be accounted for if the radiation risk for an organism as a whole, such as a human being, is to be represented. This is achieved through the concept of effective dose, where the equivalent dose is weighted for individual tissue radiosensitivity. These weighted doses can be summed to provide a total-body dose. The effective dose (E) is thus represented by the following equation:
where HT is the equivalent dose in a tissue or organ (T) and wT is the weighting factor for the tissue or organ.
2.3 Radiation health effects, standards and guidelines
An international network of scientific and regulatory bodies focuses on the protection of humans and the environment from radiation and radionuclides (see figure 3). The scientific basis is provided by the United Nations Scientific Committee on the Effects of Atomic Radiation (UNSCEAR). UNSCEAR, formed in 1955, is the official independent international authority on the sources and effects of ionizing radiation. UNSCEAR publishes reports on its assessment of the state of the latest science related to radiation sources and their potential risks to human health and the environment. The U.S. National Academy of Science plays a similar role through its periodic evaluations of the biological effects of ionizing radiation (BEIR), the latest of which is BEIR VII 2006.
The science from UNSCEAR feeds directly into the activities of the International Commission on Radiological Protection (ICRP), the goals of which are to prevent cancer and other diseases and effects associated with exposure to ionizing radiation, and to protect the environment. The ICRP developed, maintains and elaborates the International System of Radiological Protection (ISRP), which is used worldwide as the common basis for radiological protection standards, legislation, guidelines, programs and practices applied to industrial and medical applications of nuclear technology and materials. This radiation protection system is based on the current understanding of the science of radiation exposures and effects as well as socio-economic factors. The ICRP is an independent international not-for-profit organization consisting of members from approximately 30 countries encompassing six continents. Funding is provided by organizations with an interest in radiological protection. The scientific secretariat is hosted in Canada. The U.S. National Council on Radiation Protection and Measurements (NCRP) serves a similar role as the ICRP.
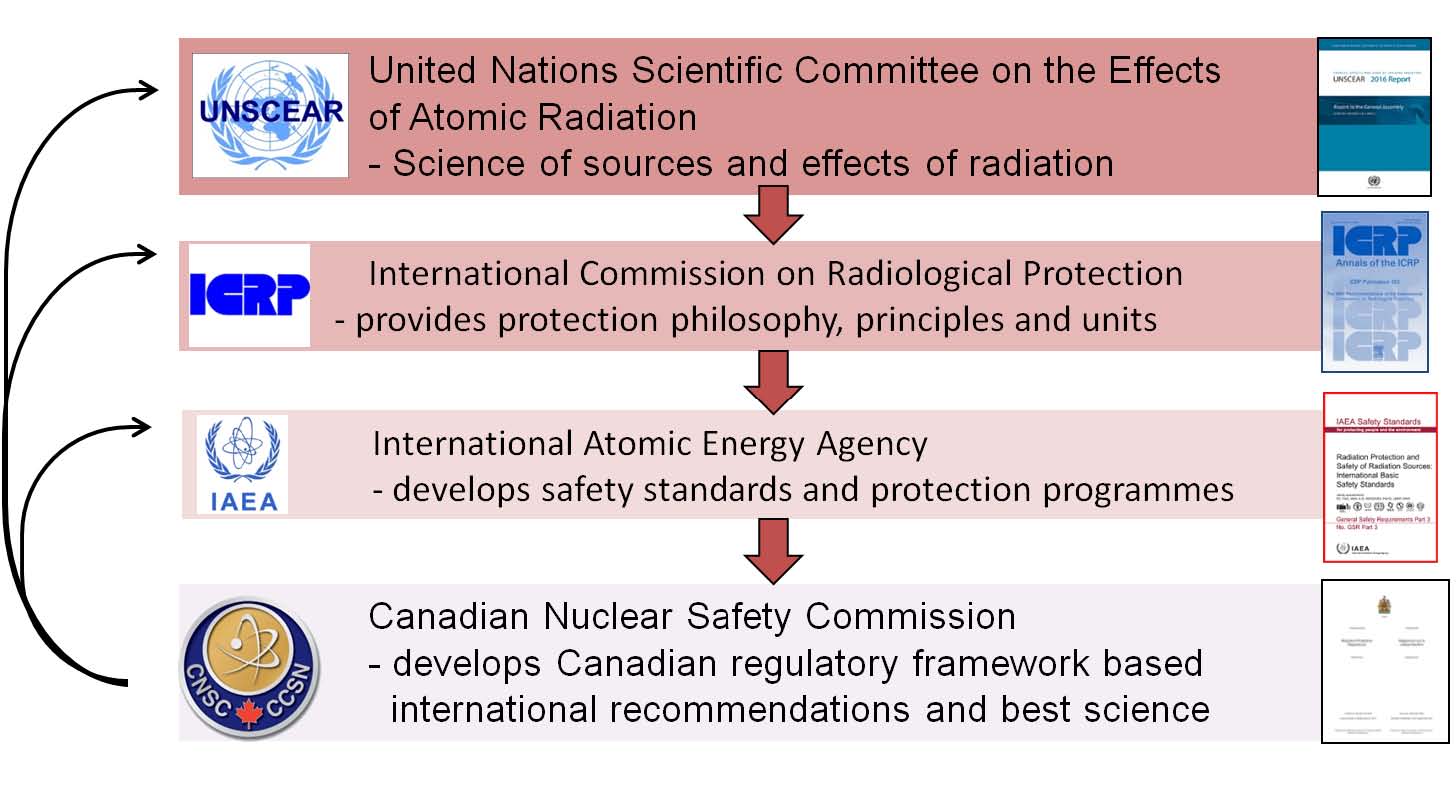
Descriptive text for Figure 3
Figure 3. This figure is a visual representation of the text discussed in section 2.3. It shows how science from the United Nations Scientific Committee on the Effects of Atomic Radiation feeds into the activities of the International Commission on Radiological Protection, which in turn provides information to the International Atomic Energy Agency whose standards and programs inform the Canadian Nuclear Safety Commissions regulatory framework. The figure also shows via arrows that the CNSC feeds relevant information back into each of these organizations
The next agency within this international network is the International Atomic Energy Agency (IAEA). This United Nations body develops safety standards and protection programs in support of the safe, secure and peaceful application of nuclear technologies for its member states. The IAEA addresses a wide range of civilian nuclear activities including the operation of nuclear installations, the production, transport and use of radioactive material, and the management of radioactive waste.
The IAEA Safety Standards consists of three sets of publications: Safety Fundamentals, Safety Requirements and Safety Guides. The first of these three establishes the fundamental safety objective and principles of protection and safety. The second sets out the requirements that must be met to ensure the protection of people and the environment, both now and in the future (e.g., is General Safety Requirement Part 3 Radiation Protection and Safety of Radiation Sources which incorporates the ISRP developed by the ICRP). Lastly, the Safety Guides provide recommendations and guidance on how to comply with the requirements.
The CNSC uses the information from this international network to develop Canada's regulatory framework. In turn, Canada financially supports the activities of these organizations, and CNSC regulatory and scientific staff participate in the work of these organizations.
These agencies have been integral in the development of the international and national radiation health science and safety frameworks discussed in section 5.
2.3.1 Effects of radiation on human health
“Today, we know more about the sources and effects of exposure to radiation than to almost any other hazardous agent, and the scientific community is constantly updating and analyzing its knowledge” (United Nations Environment Program (UNEP) 2016). As a result of more than 100 years of radiation research, the relationship between radiation exposure and specific health effects is well understood. In recent decades, science has made significant advances towards understanding the underlying mechanisms associated with exposure and effects (UNSCEAR 2015).
Such understanding is informed by a wide range of sources and scientific studies. At one end of the scale are studies of whole populations (i.e., epidemiological studies) that consider these groups' lifespans. These include the lifespan studies of the 86,500 survivors of the Second World War atomic bombing in Japan and studies of occupational exposures (routine and accidental) and accidental public exposures (e.g., Chernobyl, Goiânia). At the opposite end of the scale are the more traditional biomedical laboratory studies involving experimental animals or animal and human cell cultures. A more specific source of extensive biomedical information is studies for, and follow-up from, the extensive use of radiation and radionuclides in radiation therapy and nuclear imaging.
Radiation effects can be broadly categorized into deterministic and stochastic. Deterministic effects result when a cell receives a large enough dose such that it dies or is damaged beyond repair. The effects vary according to the radiation dose and the exposed tissue type, and effects may become evident within days to a year. These can range from reduced blood cell production (~0.5 Gy), sterility (~3–6 Gy), skin reddening and burns (<3–10 Gy), loss of gastrointestinal mucosa lining (~6 Gy) and, in extreme cases, death (> 1 Gy, although the likelihood of mortality is related to the availability of medical intervention and the dose received). These health effects are associated with the upper ranges of radiation exposure with no evident effects arising unless some threshold (approx. 0.5 Gy) is exceeded. The severity of the effect increases with increasing dose above the threshold.
Stochastic effects are associated with the modification of a cell's genetic material which is not fatal to the cell. If such damage is not adequately repaired, the resultant mutation may be replicated in cells arising from subsequent cell division. Depending on the mutation, these cells may have no discernable health effect or may lead to solid cancers and leukaemia in later years. An increase in the frequency of occurrence of heritable disease from radiation exposure has been demonstrated in animal and plant studies at moderate or high doses; however, they have not been demonstrated in human populations exposed to radiation in any range (UNSCEAR 2015). While the probability of occurrence of stochastic effects (e.g., cancer) within a population has been shown to be dependent on the dose, the severity of the effect is not dose dependent. In addition, there is generally a significant lag time or latency in the order of months to years between exposure and discernable health effects.
UNSCEAR classifies radiation exposure into the general dose bands shown in table 1.
Terminology for dose bands | Absorbed dose | Examples with associated approximation of effective dose in sieverts |
---|---|---|
High | Greater than about 1 Gy |
Severe radiation accident (e.g., firemen during Chernobyl accident)
|
Moderate | ∼ 100 mGy to ∼ 1 Gy |
Post-accident recovery operation workers at Chernobyl
|
Low | ∼ 10 mGy to ∼ 100 mGy |
Receiving multiple computer tomography scans
|
Very low | < ∼ 10 mGy |
Conventional radiography (e.g., X-rays)
|
As this assessment addresses levels of radiation in the environment and exposure of the public and non-human biota, the focus is on the “very low” range of exposures. Thus, only stochastic effects are relevant with the effect of primary interest represented by the potential for increased cancer risk.
At doses below 100 mSv/year, observed cancer incidence in people is not statistically different from zero (Health Physics Society (HPS) 2016). In other words, within the low to very low dose ranges associated with typical environmental and occupational exposures, it is not possible to determine if these exposures result in an increase in cancer incidence above the natural cancer rate. However, studies at the cellular and molecular levels demonstrate that there are biological responses (both negative and positive) at low and very low doses of radiation. The implications of these responses at the whole organ, individual person or population level are currently not known. Therefore, as a precautionary measure, for radiation protection purposes, it is necessary to mathematically estimate the risk of cancer at these low doses (i.e., < 100 mSv/year). Of the four proposed dose models shown in figure 4, the linear-no-threshold model (LNT) has been endorsed for radiation protection purposes by the ICRP and the NCRP.
The LNT extrapolates cancer risk determined from epidemiological studies of populations exposed to moderate and high doses to lower doses where effects cannot be distinguished from background. It assumes that the linear relationship present at doses of > 100 mSv/year continues down through the low to very low dose ranges. In other words, as the radiation dose increases, the risk of excess cancer proportionally increases.
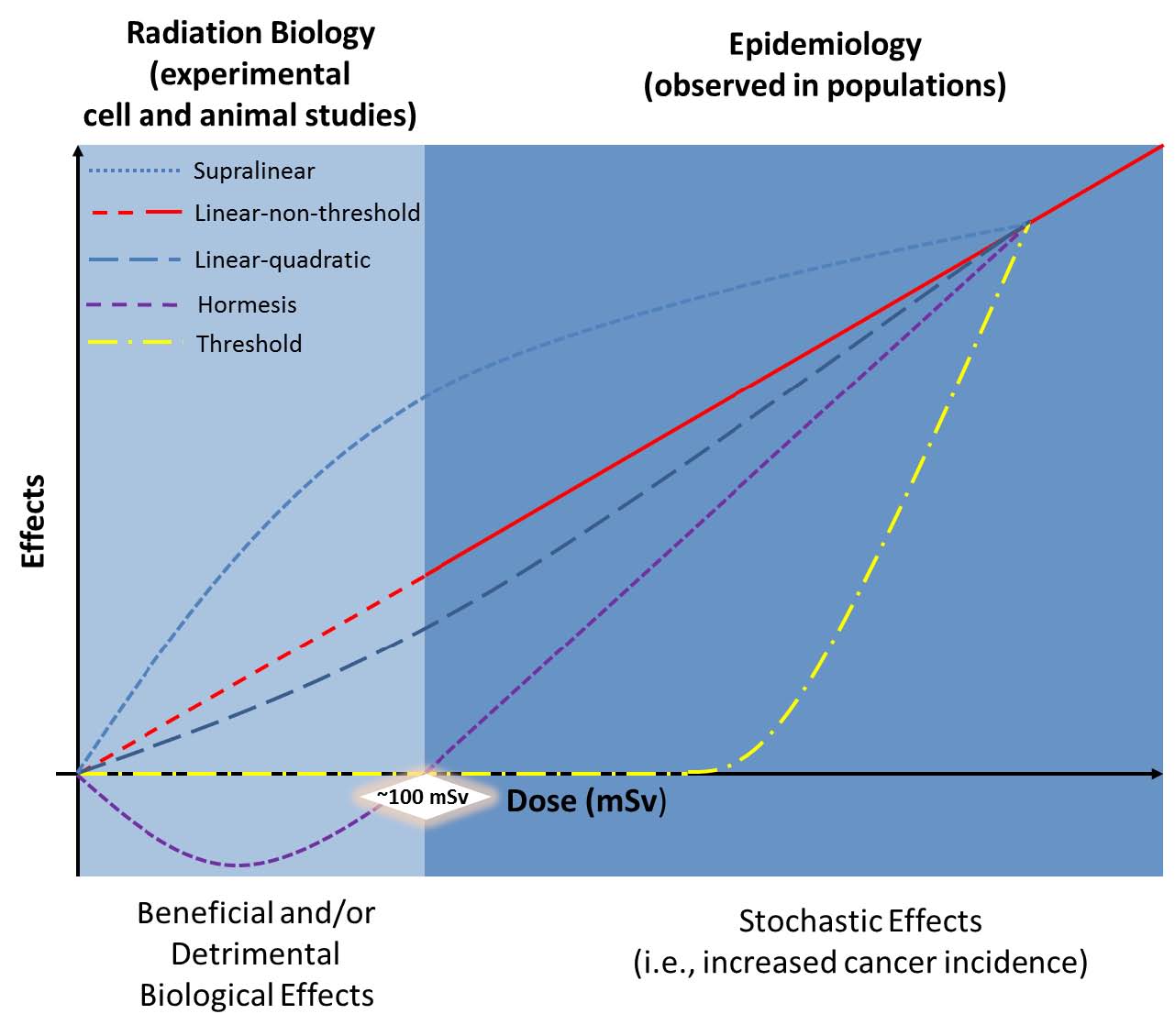
Descriptive text for Figure 4
Figure 4 is a line graph with dose in (mSv) along the horizontal axis and effects along the vertical axis. The figure is divided vertically at 100 mSv, the area below 100 mSv represents the doses where beneficial and/or detrimental biological effects have been observed through experimental cell and animal studies. The area above 100 mSv represents stochastic effects observed through population studies. The figure includes 5 data series representing different dose response models, these are:
- Supralinear: Effect increases in a linear manner quickly approaching approximately 50 mSv after which effects transition to a shallower positive linear trend
- Linea-non-threshold: effects increase in a standard linear manner
- Linear quadratic: effects increase in a shallow trend which increases slightly in steepness after exceeding 100mSv
- Hormesis: effects decrease from 0 mSv to approximately 50 mSv where they reach their minimum value, implying positive effects. After 50 mSv the trend increases back to 0 effects at approximately 100mSv, effects then increase in a linear manner beyond 100 mSv
- Threshold: 0 effects are observed until beyond 100 mSv where after which a sharp linear increase is observed
The adoption of this model has been incorrectly interpreted by some as supporting the statement that, “there is no level of radionuclides below which exposure can be defined as ‘safe;' therefore, very low levels of exposure can be significant” (CELA 2016). This is a significant over-simplification of the risks. The application of the LNT represents regulatory application of the precautionary principle. It should not be interpreted as stating that low dose exposures are unsafe.
A more accurate summation of radiation health risk is shown in figure 5. At doses below 100 mSv, the observed radiation effects in people are not statistically different from zero (HPS 2016). In other words, health effects such as cancer are biologically plausible at the low and very low dose bands, but any possible increases in carcinogenesis are so small as to be negligible and not possible to discern from natural incidence rates. This scientific information should be kept in mind when evaluating environmental exposures. This is especially important when these exposures are below the regulatory dose limit of 1 mSv/year for members of the public and represent small fractions of natural and medical diagnostic radiation exposures.
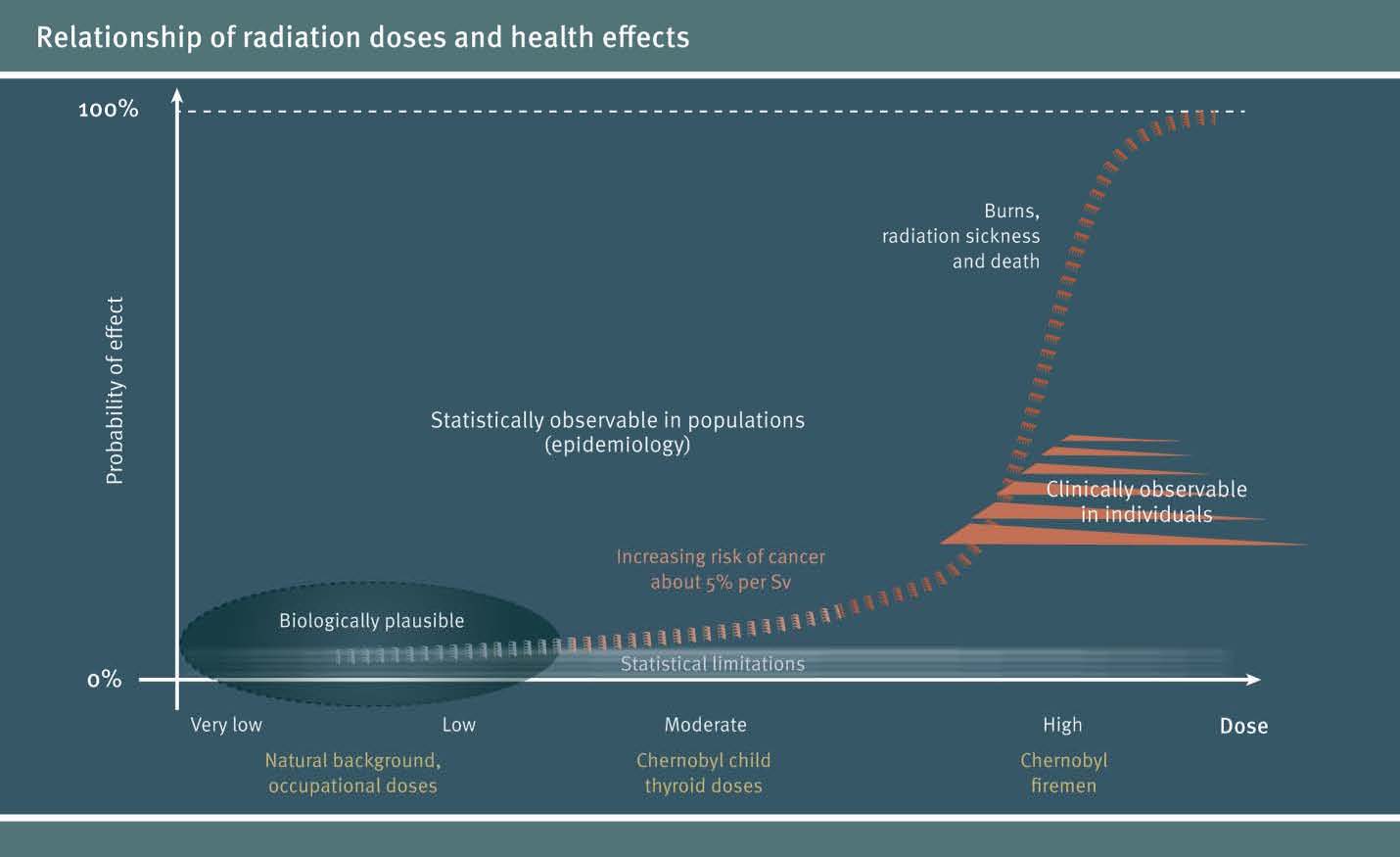
Descriptive text for Figure 5
Figure 5 shows a graphical relationship of radiation dose and health effects. The vertical axis represents the probability of an effect from 0 to 100% while the horizontal represents dose. Dose ranges from very low to low dose, representative of background radiation or occupational doses to moderate dose, representative of the doses to the thyroids of children around the Chernobyl incident; to high dose, representative of the dose firemen battling the fire from the Chernobyl incident received. Between very low to low doses effects are biologically plausible but the probability of an effect is close to zero, probability of effect slowly increases as it approaches moderate dose, at this point risk of cancer increases by approximately 5% per Sievert assuming the LNT dose response model. Between moderate and high dose the probability of clinically observable deterministic effects result (radiation burns, radiation sickness, and death) increases exponentially.
2.3.2 Radiation health standards and regulatory limits
Most international regulatory bodies as well as the CNSC adopt the radiation protection standards proposed by the ICRP. The ICRP and associated Canadian dose limits are derived from models incorporating the LNT as recommended by UNSCEAR. The Canadian Radiation Protection Regulations restrict nuclear energy worker exposures to a maximum effective dose of 50 mSv in a year and no more than 100 mSv over a five-year period. For a member of the public, the maximum dose limit is 1 mSv/year. In addition to meeting these dose limits, licensed nuclear facilities in Canada are required to keep doses as low as reasonably achievable (ALARA) to further decrease exposures. It is important when applying these dose limits, especially the public dose limit, to remember that these apply to anthropogenic doses, not natural background doses. Thus, these doses can be thought of as being incremental to natural background.
Health Canada (HC) drinking water guidelines (HC 2009) have been calculated such that consuming two litres of water per day for one year results in an effective dose of 0.1 mSv for each radionuclide taken separately. The resulting maximum allowable concentrations are available for a range of radionuclides and applied in this assessment. As uranium is more chemically toxic than it is radiologically toxic, the total uranium drinking water guideline of 20 µg/L is applied in this assessment.
The CNSC requires licensed facilities to calculate the annual doses to members of the public using their release data and the results of their environmental monitoring programs incorporating multiple exposure pathways. These are calculated as per methods approved by the CNSC which are based on the methodology identified in the Canadian Standards Association (CSA) document N288.1, Guidelines for calculating derived release limits for radioactive material in airborne and liquid effluents for normal operation of nuclear facilities (CSA 2014a). Public radiation doses from nuclear activities and facilities are required to be below the public dose limit of 1 mSv/year.
Simplified single media screening criteria have been developed by the CNSC and are used for screening radionuclide activity concentrations for single sample media in the CNSC's Independent Environmental Monitoring Program (IEMP). The CNSC screening level for fish consumption is used in this report with the derivation methodology provided in appendix A.
2.3.3 Radiological protection of non-human biota
The field of radioecology has advanced tremendously since the 1990s. Regulatory standards and guidance for environmental protection in the context of exposure of non-human biota to radionuclides have advanced in parallel. Some uncertainties remain in an academic sense, but confident statements can now be made about the absence of significant effects on populations or ecosystems at the low levels of radionuclides that typically result from the operation of Canada's nuclear facilities. More recent evidence from field monitoring of nuclear accidents at Chernobyl and Fukushima has also clarified whether or not large-scale effects occur at very high levels of contamination. The evidence for population level effects remains weak.
Most major developments in the science of radiological protection for non-human biota have been the result of many multidisciplinary projects that began in 2000 such as: the Framework for the Assessment of Environmental Impact (FASSETT), Environmental Risk from Ionising Contaminants: Assessment and Management (ERICA), Protection of the environment from ionising radiation in a regulatory context (PROTECT), Strategy for Allied Radioecology (STAR), and Coordination and implementation of a pan-Europe instrument for radioecology (COMET). These large efforts continue to make major contributions to the scientific literature. In parallel, ICRP formed Committee 5 (Larsson 2016) to provide advice on a complete system of environmental protection for biota. This is being applied gradually. Certain countries such as the U.S., Canada and the United Kingdom have also developed national approaches and continue to refine how radioecological risk assessment is done. The necessary tools and databases to do these assessments are now very sophisticated and include dose modelling software (e.g., European Union ERICA, U.S. RESRAD-BIOTA), IAEA handbook of parameter values (IAEA 2014a), and Canadian guidance such as CSA N288.1 (CSA 2014a) and CSA N288.6, Environmental risk assessments at class I nuclear facilities and uranium mines and mills (CSA 2012).
Canada was the first nation to directly incorporate the protection of the environment into nuclear legislation. This was done within the Nuclear Safety and Control Act (NSCA) in 2000. The principle is now incorporated throughout regulations, regulatory documents and standards associated with the CNSC’s regulatory framework. A series of nuclear environmental protection standards (i.e., the CSA’s N288 series) has been developed for, and are incorporated into, CNSC regulatory documents and licences. Similarly, the IAEA has been actively facilitating international collaboration in science and modelling through the multi-year Environmental Modelling for Radiation Safety and Modelling and Data for Radiological Impact Assessments projects. With these many significant developments, environmental protection of non-human biota in the nuclear sector is now a fundamental requirement of several governments (e.g., UK Environment Agency 2010), and has been incorporated in the Basic Safety Standards of the IAEA (IAEA 2014b) and the European Union (CEU 2013). To date, Canada is the only country in which doses of radiation to non-human biota and radiological risks to these organisms are used by the nuclear regulator for decision making.
Altogether, a great deal is known about the effects that can be anticipated under different circumstances and how to manage the impacts of operating facilities to minimize any potential impacts.
The specific screening benchmarks for radiological doses are discussed in section 4 where a generic screening risk assessment is presented for Great Lakes aquatic biota.
3.0 Radionuclides in the Great Lakes
Radionuclides within the Great Lakes are of natural or anthropogenic origin. Radionuclides of natural origin are composed of two main groups: cosmogenic radionuclides (i.e., those that are formed by the interaction of cosmic radiation with nuclei present in the atmosphere) and terrestrial radionuclides (i.e., those present in the earth's crust and in all environmental media). The latter consist of non-chain radionuclides with half-lives comparable to the age of the earth or those which are progeny of three chains of radioactive elements: uranium-238, thorium-232 and uranium-235.
Anthropogenic sources of radionuclides to the Great Lakes can be subdivided into weapons-testing fallout and industrial activities. Some industrial activities can increase the release of natural radionuclides. The more significant contributors among these include resource extraction (e.g., oil and gas, coal, phosphate and uranium), uranium refining and conversion, and coal thermal energy production. Other nuclear activities, such as nuclear power generation and nuclear medicine, release anthropogenic radionuclides.
3.1 Natural background and weapons testing fallout
Worldwide, the main cosmogenic radionuclides contributing to doses are carbon-14, sodium-22, beryllium-7 and tritium. Of these, carbon-14 and tritium are of most interest to the Great Lakes as natural activity concentrations can be further augmented by anthropogenic releases arising from weapons fallout and nuclear power plants (NPPs). Tritium is the most relevant as it accounts for more than 99 percent of total radioactivity released from Canadian CANada Deuterium Uranium (CANDU) reactors.
Since 1959, HC has continuously operated a network of stations across the country to monitor radioactivity levels in the environment. The original purpose of the network was to measure radioactive fallout from intensive nuclear weapons testing during the Cold War. With the growth of nuclear power in Canada in the 1970s, the network was expanded to include monitoring of radioactivity levels in the vicinities of the nuclear power stations. This monitoring continues today (see section 5.3.3).
Despite the post 1970s surge in civilian nuclear activities, including such accident events as Chernobyl and Fukushima, the past use and testing of nuclear weapons still dominate the total anthropogenic radioactivity present in the environment (HC 2015). The significance of bomb testing to airborne exposure is demonstrated in figure 6. Atmospheric cesium-137 peaked in the early 1960s. Relative to the bomb releases, the operation of nuclear power plants and both the Chernobyl and Fukushima accidents have had little influence on North American atmospheric radioactivity.
The systematic monitoring of the Great Lakes water for radionuclides under the aegis of the GLWQA commenced in 1973. The focus of this monitoring was on weapons-testing related radionuclides and those associated with releases from NPPs. Selected radionuclides included plutonium-239, plutonium-240, strontium, tritium and cesium. Numerous papers and reports published by Canadian and U.S. federal, provincial or state agencies (IJC 1983), the power generation industry (Ontario Hydro 1987), and academia were reviewed and summarized by Joshi (1991). These studies confirmed the presence of radionuclides in the Great Lakes basin from a mix of natural (i.e., cosmogenic and terrestrial) and anthropogenic sources.
Studies confirm that for most anthropogenic radionuclides, levels currently present in the Great Lakes have decreased relative to the peaks in the 1960s. This is despite the commencement of operation of Canada's fleet of NPPs in the 1970s. In other words, radionuclide concentrations have decreased in the Great Lakes over the period of operation of the Canadian nuclear power plants. This is illustrated by comparing 1970s radioactivity levels influenced by peak atmospheric bomb testing period and 1980s monitoring in the post atmospheric bomb testing period (see table 2).
Location | Cesium-137 | Plutoniun-239, 240 | Strontium-90 | Tritium | ||||
---|---|---|---|---|---|---|---|---|
1973 | 1981 | 1973 | 1981 | 1973 | 1981 | 1973 | 1981 | |
Drinking water guideline | 10 | 0.5 | 5 | 7,000 | ||||
Lake Ontario | 0.0018 | 0.0008 | 0.00000024 | 0.00000017 | 0.0466 | 0.0247 | 11.1 | 13.5 |
Lake Erie | 0.0008 | 0.0006 | 0.0000002 | 0.00000018 | 0.0407 | 0.0235 | 12.6 | 8.5 |
Lake Huron | 0.0015 | 0.0012 | 0.00000062 | 0.00000048 | 0.0318 | 0.0226 | - |
10.6 |
Lake Michigan | 0.0018 | 0.0012 | 0.00000077 | 0.00000044 | 0.0305 | 0.0171 | - |
7.4 |
Lake Superior | 0.0028 | 0.0017 | 0.00000022 | - |
0.0194 | 0.0163 | 11.1 | 6.7 |
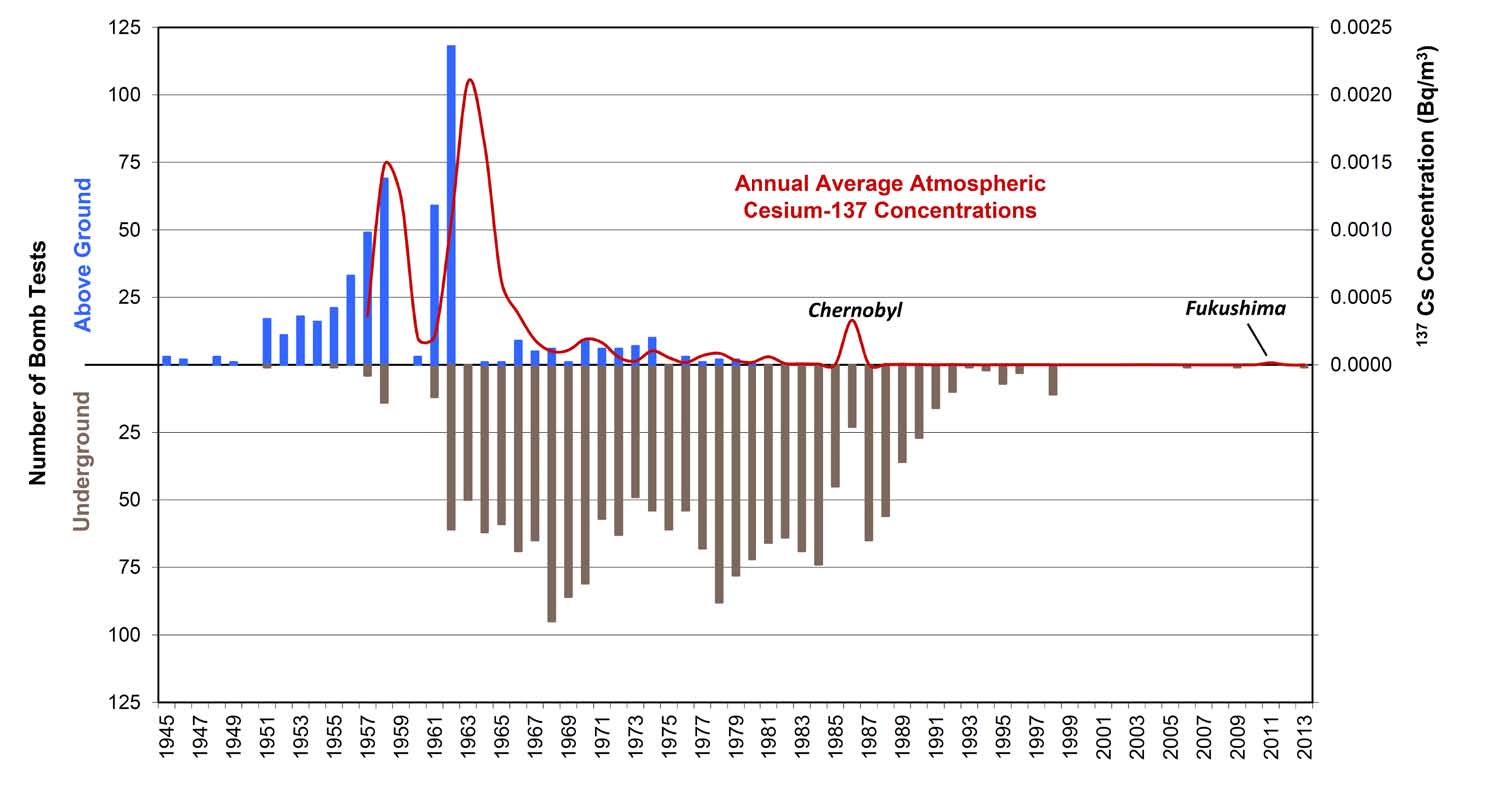
Descriptive text for Figure 6
Figure 6 shows the number of nuclear weapon tests carried out above and below ground globally on an annual scale between 1945 and 2013. The graph also plots atmospheric Cs-137 concentration from 1957 to 2013. From 1945 above ground tests increase until reaching a peak of approximately 120 tests in 1962 before falling sharply and decreasing to 0 tests in the early 1980s. The number of underground tests is relatively low until 1962 where there is a sharp increase from fewer than 10 tests in 1961 to over 50 in 1962. The number of underground tests annually stays relatively constant until the late 1980s where it begins to fall, approaching 0 in the early 2000's. Atmospheric Cs-137 follows the number of atmospheric nuclear tests fairly closely on a lag of about a year, reaching its peak in approximately 1964 of 0.0021 Bq/m3. With the discontinuation of atmospheric nuclear tests the atmospheric concentration of Cs-137 falls quickly towards 0 throughout the 1960's and 70's. There is a small but significant spike to approximately 0.0003 Bq/m3 around 1987 which coincides with the Chernobyl incident, after which Cs-137 in the atmosphere decreases towards 0, with a minute spike in 2011 coinciding with the Fukushima incident.
Between these two sampling periods, activities of most radionuclides declined at a rate of 2–5% percent per year (Joshi 1991). Activities of other short-lived anthropogenic radionuclides (e.g. cerium-144 with a half-life of 284 days and antimony-125 with a half-life of 2.8 years) were originally detected at extremely low levels and expected to decrease over time, primarily due to decreased fallout as a result of the 1963 ban on atmospheric weapons testing (Joshi 1991). Indeed, sampling completed by Baweja et al. (1987) from 1981 to 1984 in the Niagara and St. Lawrence rivers showed lower numbers for tritium (averaging ∼ 5–8 Bq/L) likely due to whole lake dilution while antimony-125 (Sb-125) averaged at or below the detection limit as a result of both dilution and its short half-life.
Both Baweja et al. (1987) and Joshi (1991) concluded that radionuclide concentrations in the Great Lakes waters were low. They also noted that radiological doses committed from ingestion of surface waters were below the dose of 10 µSv/year as stipulated in the GLWQA at that time.
Tritium fallout vs CANDU releases
The historical and current monitoring data set indicates tritium in the Great Lakes is the radionuclide most influenced by anthropogenic sources (i.e., nuclear weapons testing fallout and CANDU reactors).
Figure 7 shows the average lake-wide tritium concentrations complied from several sources between 1953 and 1997 along with the results of the most recent 2008 sampling campaigns completed in conjunction with the Canada Centre for Inland Waters (King-Sharp 2009), which is responsible for the data management and field support of Environment and Climate Change Canada's Great Lakes Surveillance Program. Consistent with the work of Joshi (1991), the maximum concentrations of tritium in all of the Great Lakes peaked prior to the operation of Canada's NPPs, and tritium concentrations have decreased continuously despite the operation of Canada's NPPs.
Determining the relative contribution of natural background, atmospheric bomb testing and CANDU releases to the Great Lakes has been the objective the Great Lakes Tritium Model developed to support public dose calculations associated with Canadian licensed NPPs (Klukas 1999, King-Sharp 2009).
The model was developed through the compilation of the historical monitoring data set that began in 1958 and periodic sampling campaigns completed for updating and testing the model. Model predictions have been tested against whole-lake surveys completed in 1991, 1997 and 2008. The model has performed well, with the ratio of predicted to observed tritium concentrations being 1.35, 1.15 and 1.1 for Lake Huron and 1.14, 1.11, and 1.21 for Lake Ontario. The results of the 2008 survey are consistent with this pattern. They show a clear decline in average lake-wide tritium concentrations as weapons-sourced tritium decays unmasking a small (few Becquerels) but evident tritium signature from Lake Huron through Lake Ontario primarily associated with controlled liquid effluent releases from CANDU reactors (see figure 7).
The performance of the model – which is regarded to be relatively accurate but slightly over-predictive – helps to predict future Great Lakes water quality. Figure 8 presents model predictions from 1953 to 2038 for Lake Huron and Lake Ontario. The results clearly demonstrate what the empirical field measurements confirm: that tritium levels today, outside the immediate vicinity of NPP discharge points, are significantly lower than those experienced from the 1960s through the 1990s, despite CANDU inputs. Projecting forward to 2038 shows the relative contribution from CANDU releases increasing as weapons fallout continues to decline due to decay. Total concentrations are predicted to stabilize at approximately 5 Bq/L from roughly 2000 onward. This is supported by the results of Ontario drinking water supply monitoring program (see section 5.3.2) and the NPPs' reference surface water monitoring programs (see section 3.2.4). Compilation of this monitoring data results in a mean tritium concentrations of 3.1 (± 0.087 SD) Bq/L with a maximum recorded value of 5.6 Bq/L.
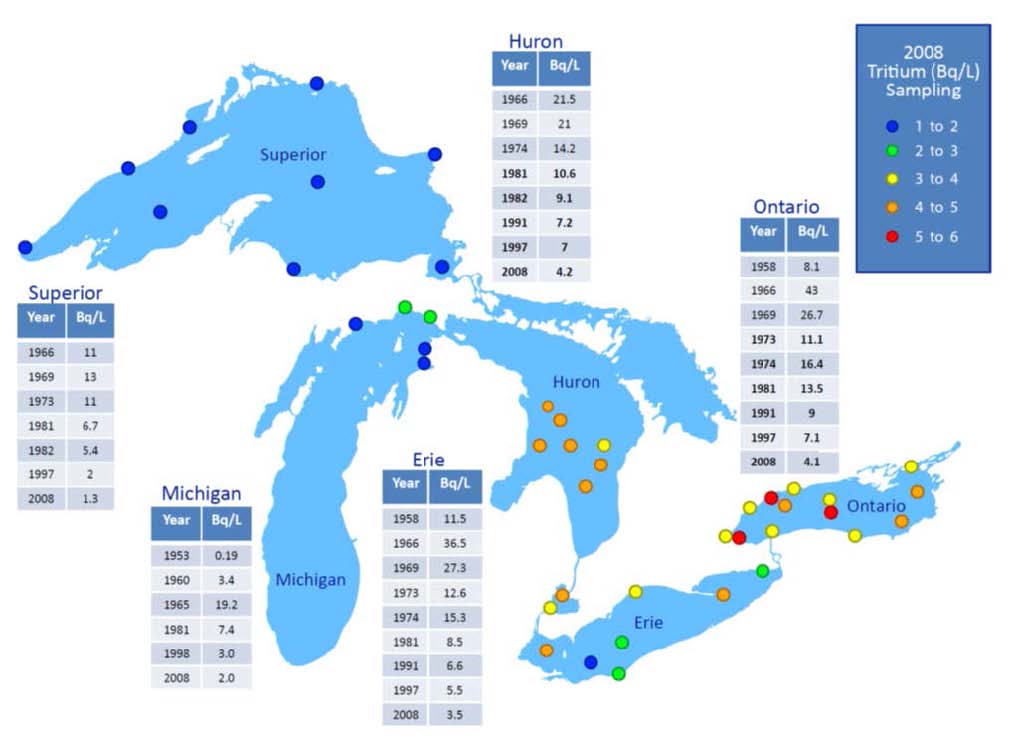
Descriptive text for Figure 7
Figure 7 displays a map of the great lakes along with tritium sampling locations for the most recent 2008 sampling campaign. These sampling locations are colour coded according to the concentration of tritium from that location. Lake Superior has the lowest concentration of tritium through time, all samples from 2008 were between 1-2 Bq/L. Lake Michigan water samples also have a low tritium concentration, ranging from 1-3 Bq/L. Tritium concentrations in Lake Huron range from 3-5 Bq/L. Tritium concentrations in Lake Erie range from 1-5 Bq/L. Tritium concentrations in Lake Ontario range from 3-6 Bq/L. The figure also contains lake specific tables of historical concentrations of each lake through time ranging from 1953 to 2008. Historically all lakes show a maximum tritium concentration in the mid to late 1960's before decreasing to low concentrations in 2008.
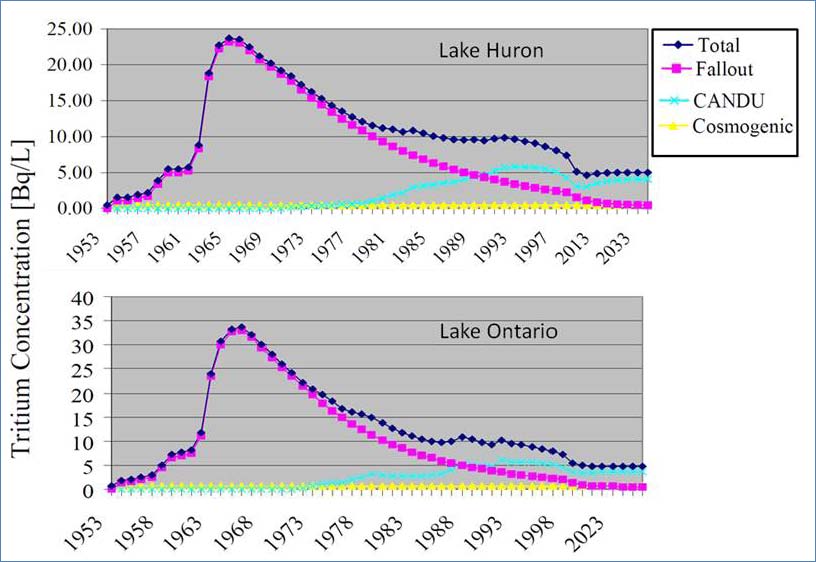
Descriptive text for Figure 8
Figure 8 consists of two line graphs, one for Lake Huron and one for Lake Ontario, showing tritium concentration in the water through time. The vertical axis refers to tritium concentration in Bq/L with the horizontal axis representing time from 1953-2038. Each graph has 4 lines with the two lakes exhibiting similar trends over time but differing slightly in magnitude for tritium concentration.
Total tritium: Begins close to 0 in 1953 before sharply increasing towards a peak in the mid-1960s, concentrations then begin to decrease uniformly until the late 1970s where the trend becomes shallower, approaching a constant of approximately 5 Bq/L
Fallout tritium: concentration increases to a peak in the late 1960s before decreasing on an ever shallowing slope to 2023
CANDU tritium: concentration is 0 until the late 1970's where it begins to increase with a shallow trend before stabilizing at just under 5 Bq/L
Cosmogenic tritium: levels remain constant through time at concentrations close to 0
In summary, the evidence indicates that tritium levels have continued to decline since the peaks in the 1960s, and despite CANDU releases. This decline can be expected to stabilize based on modelling and current water monitoring results to concentrations approximating 5 Bq/L or lower in Lake Ontario and Lake Huron. Current monitoring confirms this.
Terrestrial radionuclides
Terrestrial radionuclides include primordial radionuclides and the decay progeny of three chains of radioactive elements: uranium-238, thorium-232 and uranium-235. Worldwide, the main contributors to natural background radiation doses are potassium-40 in the body approximating 0.17 mSv/year, and the uranium-238 and thorium-232 series radionuclides, which account for approximately 0.12 mSv/year (UNSCEAR 2010). The primordial radionuclides of most relevance to the Great Lakes are those of the uranium-238 series. These enter surface waters through the weathering and erosion of minerals and soils, and can be further enhanced by releases from nuclear industrial sources such as uranium mining and milling, tailings management, fuel refining and conversion, and non-nuclear sources such as resource extraction (e.g., hydrocarbon extraction and mining of metal, coal and phosphates) and coal thermal power generation. Environmental concentrations for primordial radionuclides associated with the nuclear fuel cycle in the Great Lakes basin are addressed in section 3.2.
3.2 Industrial sources
A range of anthropogenic activities can result in releases of radionuclides to the Great Lakes including industrial, commercial, research and medical activities. Activities associated with the nuclear fuel cycle or the use of substances created via the nuclear fuel cycle are regulated in Canada by the CNSC. CNSC-regulated facilities associated with the Great Lakes basin discussed in this section are identified in figure 9. Interactive maps showing the locations for the complete range of nuclear facilities regulated by the CNSC throughout Canada are available on the CNSC's website.
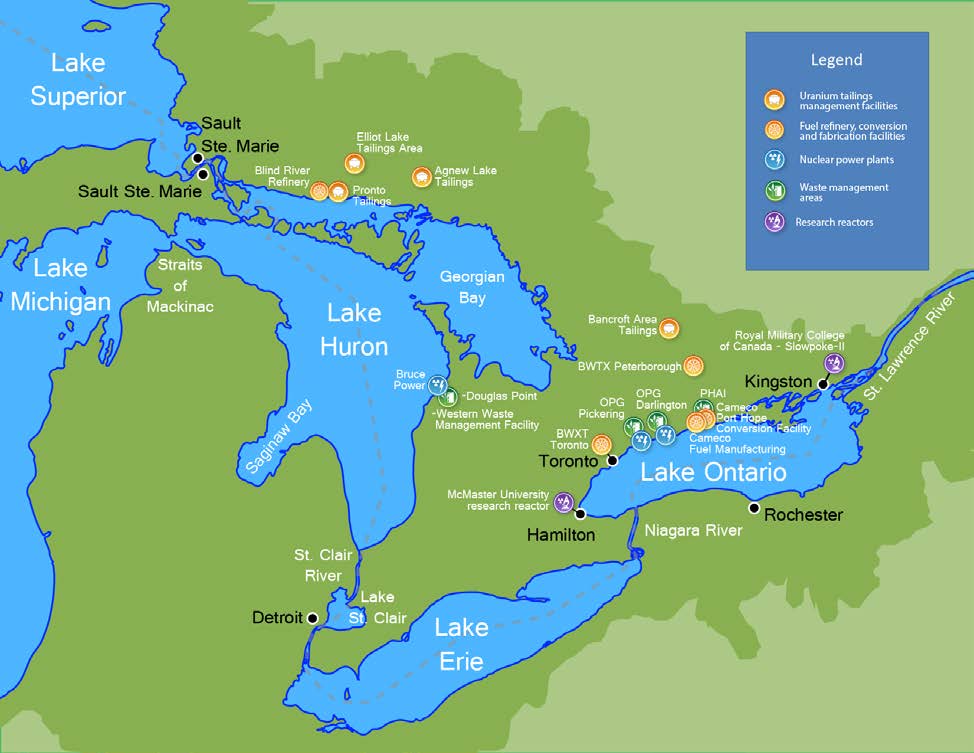
Descriptive text for Figure 9
This figure shows the location of various Canadian nuclear facilities within the Great Lakes Watershed. There are 7 facilities in close proximity to Lake Huron, a refinery and tailings facility along the northern coast and two more tailings facilities further inland. Along the eastern coast of Lake Huron there is the Bruce nuclear power generating station and two waste management areas on the same property. In south-central Ontario there is a tailings area in Bancroft as well as a processing facility in Peterborough. Along Lake Ontario's Canadian coast there are 10 nuclear facilities. Two research reactors located in Hamilton at the western end of the lake and one in Kingston along the northeastern side. There are 3 processing facilities along the northern coast: 1 in Toronto and 2 in Port hope, 2 nuclear power generating stations with accompanying waste management facilities, and a final waste management facility in Port Hope.
As a result of the regulatory oversight provided by the CNSC for nuclear fuel cycle activities, there is extensive data on releases and environmental concentrations of the most significant radionuclides associated with these activities. The following sections outline the different types of facilities that the CNSC regulates in the nuclear fuel cycle and includes a summary of the:
- overview of the facility type
- presence and location in the Great Lakes basin
- primary radionuclides of interest
- facility environmental monitoring programs
- monitoring data results and comparisons to criteria
CNSC-licensed facilities are required to have extensive environmental protection programs that address releases of nuclear (i.e., radionuclides) and hazardous substances (e.g., chemicals) (see section 5.2.1 for environmental protection regulatory requirements). This document limits its focus to radiological monitoring elements associated with the Great Lakes themselves or waters flowing into the Great Lakes.
Non-nuclear fuel cycle activities that may release radionuclides to the Great Lakes are discussed in section 3.2.8.
For information on all CNSC compliance requirements and monitoring results, the sector-specific regulatory oversight reports (RORs) may be consulted. The RORs are presented annually to the Commission at public meetings where written interventions are encouraged. Participant funding is also available upon successful application to the CNSC. These reports are available for comment prior to Commission meetings with final versions posted on the CNSC's website. In the following sections, the links to the specific RORs are provided.
To complement compliance activities, the CNSC has established its IEMP to verify that the public and environment around CNSC-regulated nuclear facilities are not adversely affected by releases to the environment. This verification is achieved through independent sampling and analysis by the CNSC. Facility specific results are available on the CNSC website. Further information on the IEMP is provided in section 5.2.2 on the regulation of nuclear activities in Canada.
3.2.1 Uranium mines and mills
The nuclear fuel cycle begins with the mining and milling of uranium ore. All of Canada's currently operating uranium mines and mills are located in the Athabasca Basin of northern Saskatchewan with no connection to the Great Lakes basin. The RORs for the uranium mining and milling sub-sector are available on the CNSC website. Uranium mining and milling has historically occurred in Ontario, with the greatest activity concentrated in the Elliot Lake region.
The Elliot Lake region supported active mining and milling for approximately 40 years. The region now contains 11 decommissioned mining operations situated within the Serpent River Watershed. This watershed ultimately flows into the north channel of Lake Huron. The monitoring program was designed to assess the recovery of the receiving environment following the implementation of decommissioning. Commenced in 1999, the program was designed to evolve in response to monitoring results, and report to a joint regulatory advisory group with representatives from multiple federal and provincial agencies. The program addressed radionuclides within the natural uranium series (i.e., the uranium-238 series) and heavy metals.
The monitoring program element of most relevance to this assessment is that of water quality nearest the outflow to Lake Huron. The results for the two stations situated in the lower reaches of the Serpent River with an extensive historical record are provided in figure 10. The original impact from the historic mining practices on water quality is evident in the early 1960s where radium-226 increased to such an extent that it briefly exceeded current drinking water guidelines. Activity levels since this peak have remained below current drinking water guidelines and have consistently declined over time returning to background levels. At no point in time have uranium concentrations at these locations exceeded the drinking water guideline nor the guideline for the protection of aquatic life and concentrations are currently at natural background levels.
On the basis of these results, it is clear that Elliot Lake activities have little influence if any on radionuclide activity levels in Lake Huron. A similar conclusion was reached by Joshi (1991) based on monitoring activities of the Province of Ontario. Therefore, this area will receive no further discussion within this report.
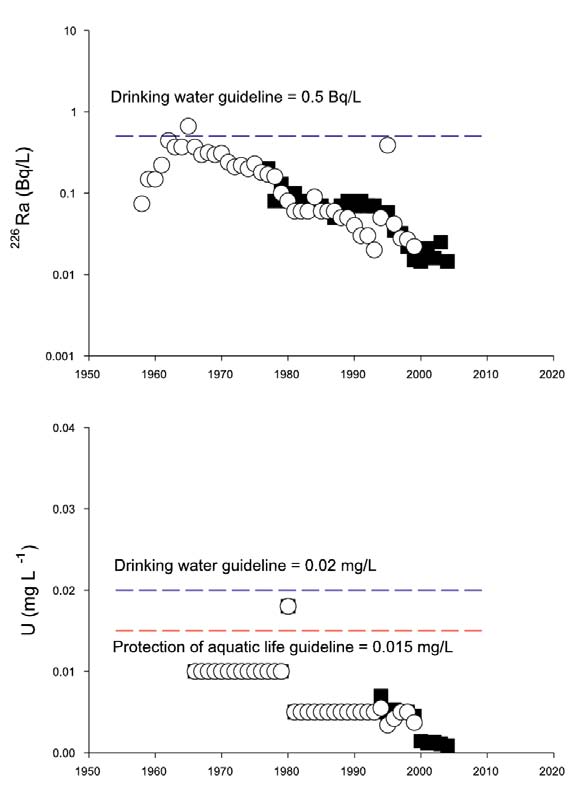
Descriptive text for Figure 10
Figure 10 consists of two figures, one displaying radium-226 concentration on a logarithmic scale and the other uranium concentrations on a linear scale. Concentrations of these two radionuclides are plotted for two sampling stations, the Serpent River and Lake McCarthy between 1960 and 2004. The graphs also show the drinking water guideline for radium-226 (0.5Bq/L) and uranium (0.02mg/L) as well as the protection of aquatic life guideline for Uranium (0.015 mg/L)
Radium concentrations in the Serpent River increase rapidly towards a peak just below 1 Bq/L in the mid-1960s after which they declined below the drinking water guideline to levels as low as approximately 0.01 Bq/L in 1999. Concentrations in McCarthy Lake reported from the 1980s to 2004 have all been below the drinking water guideline and overlap the results of the Serpent River for the similar time period.
Uranium concentrations in the Serpent River and McCarthy Lake have been at analytical method detection limits which have decreased over time. Concentrations have been below the drinking water and the aquatic life guidelines, with the exception of the 1980 reporting period which was recorded as above the aquatic life criteria but below the drinking water guideline.
3.2.2 Refining and conversion
The next step of the nuclear fuel cycle is uranium refining and conversion. There are two refining and conversion facilities on the Canadian side of the Great Lakes basin: the Blind River Refinery (BRR) and the Port Hope Conversion Facility (PHCF). The BRR is the first stage in the Canadian refining and conversion process. Materials move from the BRR to the PHCF for further processing.
The performance of both these facilities is presented to the Commission in the annual ROR for nuclear processing facilities.
Blind River Refinery
The BRR is located east of the community of Blind River on shores of the north channel of Lake Huron. The facility is bordered to the south by Lake Huron and to the west by the Mississagi River (see figure 11).
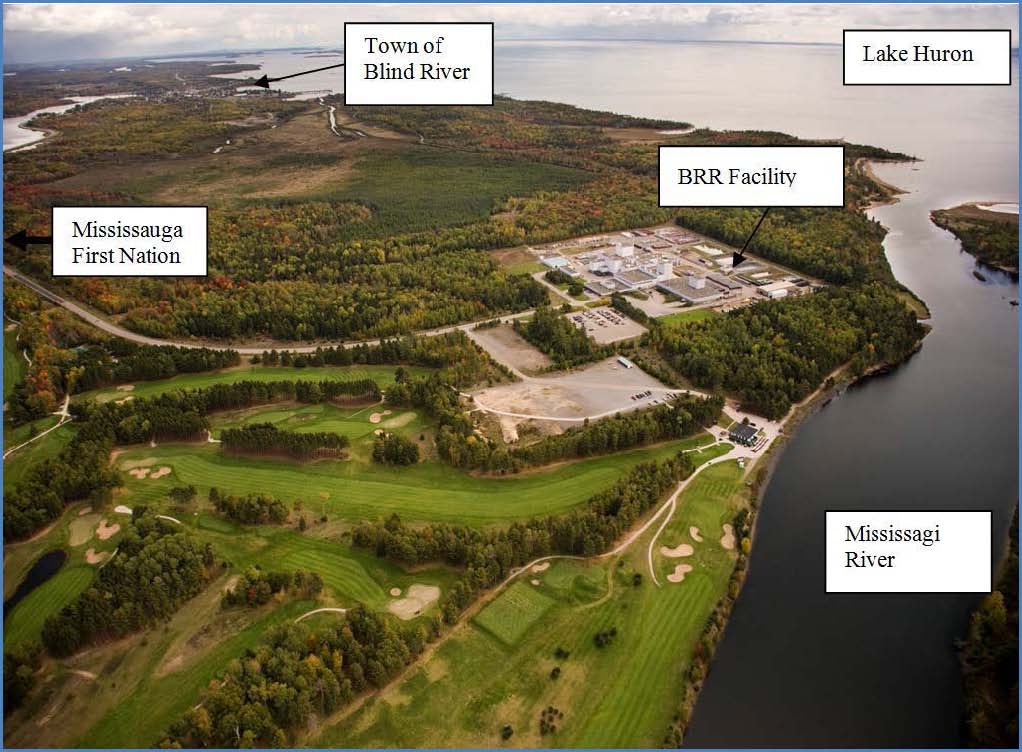
Descriptive text for Figure 11
Figure 11 is an aerial view of the Blind River refinery with several key features of the surrounding area labeled. The Blind River Refinery lies along the northern Coast of Lake Huron, just to the east of the Mississagi River. To the north of the refinery is Mississauga first nation territory, the town of Blind River is east of the refinery.
The refining and conversion process commences at the BRR, which receives uranium concentrate (e.g., yellow cake from uranium mills) and small quantities of scrap natural uranium bearing materials such as uranium dioxide and natural uranium metal. The process involves separating the uranium from impurities through a series of chemical processes to produce a high-purity uranium trioxide (UO3). The UO3 is transported for further processing to the PHCF.
BRR submits quarterly and annual compliance monitoring and performance reports to the CNSC that include the results of their environmental monitoring program. These reports are available on the Cameco website.
The BRR emissions and effluent monitoring program addresses releases to the atmosphere and discharges to surface waters. The receiving environment monitoring program includes groundwater, surface waters, ambient air and soil. Only the results of the radionuclide environmental monitoring program associated with releases to Lake Huron are summarized here.
Uranium is the primary radionuclide of interest associated with this facility. The majority of uranium decay products from the ore are retained within the mine tailings that are managed at the uranium mill sites. Monitoring therefore concentrates on uranium and radium-226. Treated process effluents are released offshore to the north channel of Lake Huron through a diffuser. Table 3 summarizes the results of Lake Huron water samples collected at a sampling station near the diffuser.
Year | Uranium (µg/L) | Radium-226 (Bq/L) | ||
---|---|---|---|---|
Annual average | Annual maximum | Annual average | Annual maximum | |
Guidelines |
Drinking water: 20 Protection of aquatic life: 15 |
Drinking water: 0.5 | ||
2006 | 0.22 | 0.31 | < 0.005 | 0.006 |
2007 | 0.2 | 0.28 | < 0.005 | < 0.005 |
2008 | 0.51 | <0.9 | 0.0006 | 0.008 |
2009 | 0.21 | 0.21 | < 0.005 | < 0.005 |
2010 | 3.1 | 8.9 | <0.008 | 0.02 |
2011 | 0.4 | 0.5 | 0.006 | 0.007 |
2012 | 0.2 | 0.3 | < 0.005 | < 0.005 |
2013 | 0.4 | 0.6 | < 0.005 | < 0.005 |
2014 | < 0.2 | < 0.2 | < 0.005 | < 0.005 |
2015 | 0.2 | 0.4 | < 0.005 | < 0.005 |
Uranium and radium-226 concentrations have consistently been well below their respective drinking water guidelines and well below the criteria for the protection of aquatic life. These results indicate that this well regulated facility is not having an impact on the Great Lakes.
Port Hope Conversion Facility
The PHCF is located on the north shore of Lake Ontario in the Municipality of Port Hope, Ontario. The facility is bounded to the south by Lake Ontario and to the east by the Port Hope Harbour. The PHCF receives uranium trioxide (UO3) for conversion to either uranium hexafluoride (UF6) or uranium dioxide (UO2). UO2 is used to manufacture fuel for nuclear power plants in Canada whereas UF6 is exported to make fuel for light-water reactors around the world.
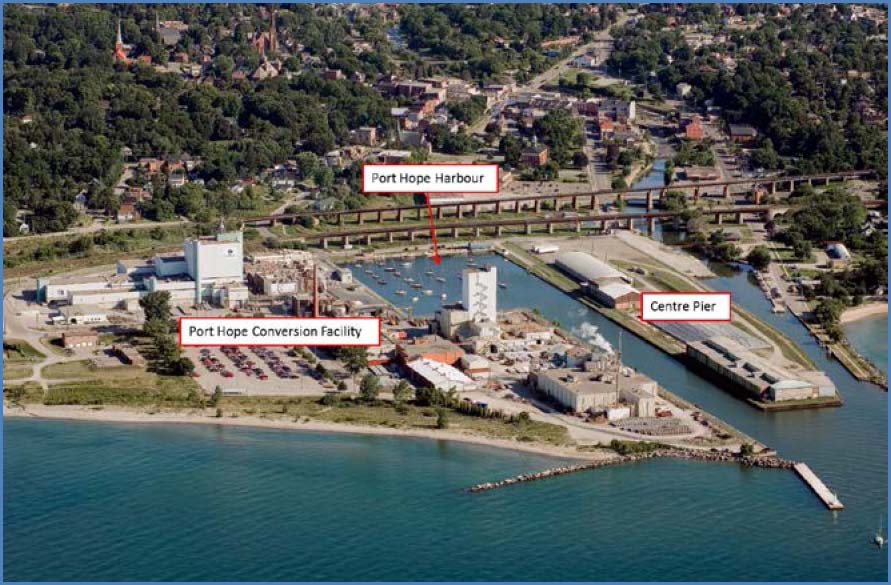
Descriptive text for Figure 12
Figure 12 is an aerial view of the Port Hope Conversion Facility and Port Hope harbour. The Port Hope Conversion Facility lies along the north coast of Lake Ontario adjacently west of the Port Hope Harbour.
The PHCF licence requires the monitoring of releases of radioactive and non-radioactive materials to the environment (i.e., to air, water, sewer and waste). Extensive environmental monitoring is also required at this facility. Once again, uranium and radium-226 are the radionuclides of interest as decay progeny have been removed along the processing chain (e.g., milling and refining). In the past, process waters were released to the harbour. In 2007, this release ceased. An evaporator was installed in the treatment system. Despite the cessation of process water releases, harbour monitoring has continued so the effects of indirect sources and the continuing legacy sources arising from contaminated groundwater, storm sewers and reflux from the contaminated sediments (see section 3.2.5 for discussion of historically contaminated harbour sediments) can be assessed. Table 4 shows the annual mean and maximum values reported from 2006 through to 2015 for uranium.
Year | Annual average (µg/L) | Annual maximum (µg/L) |
---|---|---|
Guidelines |
Drinking water: 20 Protection of aquatic life: 15 |
|
2006 | 5.5 | 16 |
2007 | 5.4 | 14 |
2008 | 6.5 | 21 |
2009 | 5.5 | 16 |
2010 | 4.4 | 21 |
2011 | 4.1 | 9.2 |
2012 | 3.7 | 10 |
2013 | 3.3 | 8.3 |
2014 | 3.3 | 7.6 |
2015 | 2.9 | 6.6 |
Despite the historical legacy of contamination, the annual average harbour uranium concentrations have consistently remained below both the drinking water and the aquatic life guidelines with a decreasing trend continuing to be evident. Even the maximum values have remained below guidelines since 2011. It should be noted, however, that the harbour is not used as a source of drinking water. With the removal of the primary source terms (i.e., contaminated sediment) by the Port Hope Area Initiative in the future, uranium in harbour waters can be expected to further decrease (see section 3.2.5).
Radium-226 has also been monitored throughout the harbour and approach channel. The majority of the samples were determined to be below the analytical detection limit reported as 0.05 Bq/L up to 2014 decreasing to 0.01 Bq/L in 2015. The maximum recorded value comes from a single sample in 2014 collected just above the sediment which measured 0.1 Bq/L. Thus, for radium-226, Port Hope Harbour waters have been consistently below the drinking water guideline for the last decade despite the legacy contamination of the sediments. In summary, the PHCF is not impairing Great Lakes water quality.
3.2.3 Fuel fabrication
At fuel fabrication facilities, the UO2 powder is pressed into small cylindrical shapes called pellets. These pellets are baked at high temperatures to gain strength and density. Afterwards, the pellets are ground to achieve a specific, precise uniform size. The pellets are then loaded into zirconium alloy tubes, which are grouped into cylindrical fuel bundles.
There are three fuel fabrication facilities in Canada which include the BWXT Nuclear Energy Canada Inc. (formerly GE Hitachi Nuclear Energy Inc.) facilities in Peterborough and Toronto, and the Cameco Fuel Manufacturing Inc. facility in Port Hope. The BWXT-owned facilities are not located on the shores of the Great Lakes.
Cameco Fuel Manufacturing is located near Lake Ontario but has no direct liquid effluent discharges to the lake. Similar to PHCF, Cameco Fuel Manufacturing is licensed to discharge small amounts of uranium in liquids to the municipal sewer. These releases report to the municipal sewage treatment plant prior to being released to the Lake Ontario via an offshore diffuser.
3.2.4 Nuclear power plants
All NPPs in Canada are CANDU reactors. These pressurized heavy-water reactors use natural uranium as fuel and heavy water as a coolant and moderator. Fission reactors in the nuclear core heat the heavy water. A heat exchanger transfers the heat from the heavy water to a light-water coolant, which powers a steam turbine with an attached electrical generator. There are three nuclear power plants located on the Great Lakes basin. Each operates a number of reactor units. The Bruce A and B nuclear generating stations are located on the shore of Lake Huron, while both Ontario Power Generation (OPG) facilities, the Pickering and Darlington nuclear generation stations, are located on the shores of Lake Ontario.
The CNSC requires all nuclear power plants to monitor and report their releases. Discharges from Bruce, Darlington and Pickering are continuously sampled and monitored. Samples are analyzed at least weekly. OPG and Bruce Power prepare quarterly operation reports to inform the CNSC. These reports contain concentrations of nuclear substances including, for each month of the quarter, the total activity or total amount released. In addition, OPG and Bruce Power submit annual reports on environmental monitoring that summarize the data from the year and compare results to historical values and to values at reference locations. The reports also present the dose calculations for the representative person for comparison to the public dose limit and to demonstrate that doses are ALARA.
Monitoring of atmospheric releases focus on the following radionuclides or radionuclide proxies (i.e., gamma, alpha or beta scans): tritium, noble gas (as a group), iodine-131, particulate gamma, particulate alpha and carbon-14. Liquid releases are monitored with focus on tritium, carbon-14 and gross beta, alpha and gamma.
Each facility is also required to have a corresponding comprehensive environmental monitoring program. The
radiological portion of this program is designed to provide empirical measurements of radionuclides within the
environmental media and sampling locations most critical to determining radiation exposure to a representative
member of the public. This person is a theoretical construct customized to capture site-specific characteristics of
the environment and public.
The programs are somewhat site-specific, but retain the same core elements. Sampling media include:
- air
- precipitation
- drinking water (i.e., from water supply plants and wells)
- aquatic samples (i.e., from water, sediments, fish and beach sands)
- agricultural products (i.e., animal feed, soils, eggs, poultry, beef, pork, milk, fruits and berries, vegetables, honey, grain and soils)
The radionuclides of interest are those known to be the primary contributors to dose. Measurements include tritium, carbon-14, iodine-131, gross beta and gamma along with external gamma dose rates at selected locations. Specifics on each facility's sampling program and the associated dose calculations can be found in the facility's annual report. These are submitted to the CNSC for review and are publicly available on the Bruce Power and Ontario Power Generation websites. RORs for NPPs can also be found on the CNSC's website.
The release data and monitoring data are used according to CSA N288.1 to calculate the public dose to ensure that no member of the public is exposed to greater than 1 mSv/year – the regulatory public dose limit in Canada. This modelling addresses multiple exposure pathways including external doses and internal doses. The calculated public dose for the highest exposed representative person is provided in figure 13 for the years 2006 through 2015. Note that doses have consistently been below 1% of the public dose limit.
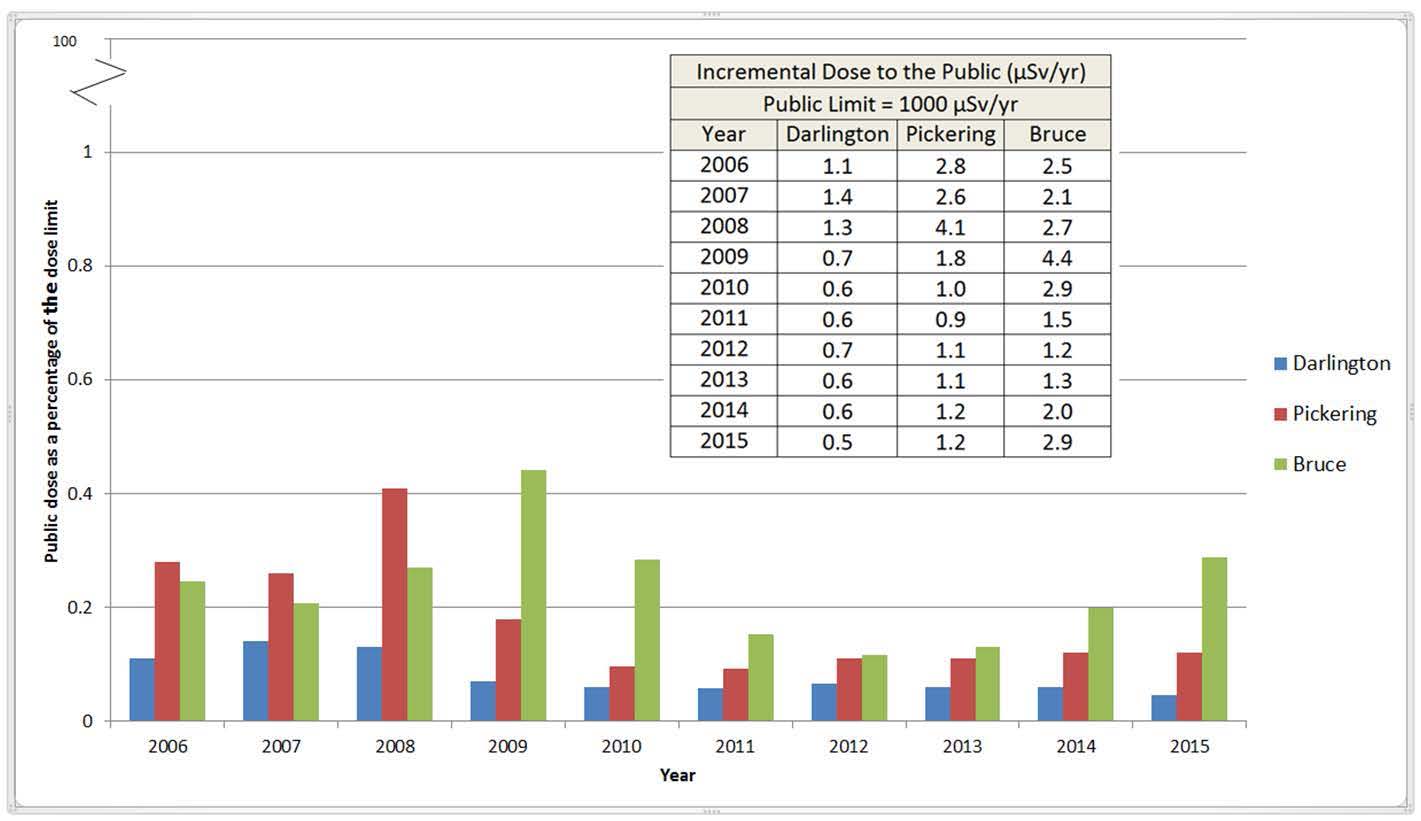
Descriptive text for Figure 12
Figure 13 is a bar graph of public dose as a percentage of the dose limit of 1mSv from 2006 to 2015 at the Darlington, Pickering, and Bruce reactor sites. Also present is an inset table displaying the incremental dose to the public in µSv/yr at the three reactor sites from 2006-2015. Between 2006 and 2015 the maximum dose as a percent of the public dose limit was calculated at the Bruce site at just over 0.4%, since then values have decreased and regularly do not exceed 0.2% of the dose limit. The inset table shows that incremental dose has been decreasing through time at the Darlington and Pickering sites and has remained fairly constant at the Bruce site with a maximum dose of 4.4 µSv/yr (as compared to the dose limit of 1000µSv/yr)
Public Limit = 1000 µSv/yr | |||
---|---|---|---|
Year | Darlington | Pickering | Bruce |
2006 | 1.1 | 2.8 | 2.5 |
2007 | 1.4 | 2.6 | 2.1 |
2008 | 1.3 | 4.1 | 2.7 |
2009 | 0.7 | 1.8 | 4.4 |
2010 | 0.6 | 1.0 | 2.9 |
2011 | 0.6 | 0.9 | 1.5 |
2012 | 0.7 | 1.1 | 1.2 |
2013 | 0.6 | 1.1 | 1.3 |
2014 | 0.6 | 1.2 | 2.0 |
2015 | 0.5 | 1.2 | 2.9 |
2016 | 0.6 | 1.5 | 1.6 |
While total dose is relevant to the overall protection of the public, the radionuclide activity concentrations in the various media can also be assessed independently against guidelines. Of specific relevance to this assessment are the results of radionuclide analyses in drinking water supply plants in close proximity to the NPPs, the intake supply for which is either Lake Ontario or Lake Huron. Intake water samples are collected either twice or three times daily and combined to form a weekly composite for tritium analyses and a monthly composite for gross beta. The results for the 10-year assessment period are summarized in table 5. The overall annual averages for this period as well as the maximum annual average are orders of magnitude below the drinking water guideline of 7,000 Bq/L. Similarly, gross beta measurements have consistently been below the drinking water guideline of 1 Bq/L.
Nuclear power plant | Water supply plant | Tritium (Bq/L) | Gross beta (Bq/L) | ||
---|---|---|---|---|---|
Annual average | Annual maximum | Annual average | Annual maximum | ||
Drinking water guideline | 7,000 | 1 | |||
Bruce | Kincardine | 6.6 | 9.8 | 0.07 | 0.08 |
Southampton | 10.8 | 14.4 | 0.08 | 0.09 | |
Port Elgin | 13.4 | 16.8 | 0.07 | 0.08 | |
Pickering | Ajax | 5.6 | 7.1 | 0.10 | 0.12 |
F.J Horgan | 4.8 | 5.8 | 0.11 | 0.11 | |
R.C Harris | 4.7 | 5.4 | 0.11 | 0.12 | |
Whitby | 5.9 | 8.2 | 0.12 | 0.19 | |
Darlington | Bowmanville | 5.2 | 6.7 | 0.11 | 0.11 |
Newcastle | 5.1 | 6.4 | 0.12 | 0.17 | |
Oshawa | 6.7 | 9.2 | 0.11 | 0.12 |
The NPPs also collect surface water samples from near-field exposure areas in Lake Ontario and Lake Huron and from further afield reference stations. There is a clear tritium signature at these near-field lake sampling stations, however, even these samples are well below drinking water guidelines (see table 6).
Nuclear power plant | Location | Tritium (Bq/L) | Gross beta (Bq/L) | ||
---|---|---|---|---|---|
Annual average | Annual maximum | Annual average | Annual maximum | ||
Drinking water guideline | 7,000 | 1 | |||
Bruce | Baie du Doré | 98.7 | 163.5 | 0.08 | 0.11 |
Inverhuron Park | 11.4 | 21.3 | 0.07 | 0.13 | |
Scott Pt. Lake | 56.2 | 99.5 | 0.07 | 0.09 | |
Pickering | Frenchman's Bay | 29.4 | 49.9 | ---1 | ---1 |
Beachfront Park | 21.7 | 41.4 | ---1 | ---1 | |
Squires Beach | 19.1 | 31.1 | ---1 | ---1 | |
Darlington | Darlington Provincial Park | 7.6 | 9.5 | 0.132 | 0.172 |
McLaughlin Bay | 24.3 | 31.4 | 0.202 | 0.222 | |
West/East Beach | 4.7 | 5.4 | 0.132 | 0.172 | |
Courtice Road Beach | 7.1 | 7.9 | ---2 | ---2 | |
1 Pickering does not monitor gross beta in surface waters. 2 Darlington stopped monitoring gross beta in lake water in 2013. Courtice Road Beach was added to the environmental monitoring program in 2013. |
Fish are also collected and analyzed for radionuclides from Lake Huron and Lake Ontario. Fish are collected in the vicinity of effluent releases and much farther away where the water quality is expected to be at background. The core fish sampling program is consistent among the three NPPs. Eight fish are collected per target speciesFootnote 3 per sampling location. Some additional fish sampling is also completed on a site-specific basis.
The complete fish database is not included in this assessment, however, the pattern of results is similar for all species. This assessment concentrates on white suckers. This fish has been the most consistently collected target species and better integrates both lake water and sediment exposure due to its foraging habits. Each replicate fish sample is analyzed for free water tritium content, carbon-14, cobalt-60, cesium-137 and potassium-40 (note potassium-40 is a significant natural dose contributor and not of NPP origin).
The results of the fish sampling program over the 10-year assessment period are shown in table 7 along with the relevant radionuclide specific CNSC screening benchmarks. Both the average and the maximum values for the radionuclides in fish flesh are orders of magnitude below CNSC screening benchmarks for human consumption (equal to 0.1 mSv per year).
Nuclear power plant | Tritium | Cesium-134 | Cesium-137 | Carbon-14 | Cobalt-60 | Potassium-40 | ||||||
---|---|---|---|---|---|---|---|---|---|---|---|---|
͞x | Max | ͞x | Max | ͞x | Max | ͞x | Max | ͞x | Max | ͞x | Max | |
CNSC screening level1 | 488,000 | 710 | 1,040 | 16,800 | 1,350 | 1,020 | ||||||
Bruce | 2.48 | 5.88 | 0.04 | 0.067 | 0.07 | 0.13 | 30.26 | 33.1 | 0.04 | 0.084 | 28.54 | 34.2 |
Pickering | 1.32 | 2.88 | 0.02 | 0.044 | 0.05 | 0.089 | 29.47 | 33.5 | 0.03 | .067 | 29.64 | 34.9 |
Darlington | 1.04 | 1.78 | 0.02 | 0.044 | 0.04 | 0.067 | 28.89 | 33.7 | 0.03 | 0.067 | 29.52 | 34.7 |
1. See appendix A for screening level derivation |
Overall, the environmental monitoring data demonstrates that despite slight increases in levels of radioactivity in close proximity to the NPPs, the public is protected. This fact is supported by the site-specific multi-pathway, multi-radionuclide exposure modelling for public dose and by the media-specific screening assessments for water supply plants, lake waters and fish tissue monitoring.
3.2.5 Waste management
This section provides an overview of radioactive waste-management activities as they relate to the Great Lakes. More information on radioactive waste management in Canada is provided on the CNSC's website.
Radioactive wastes are classified as low-, intermediate- or high-level depending on the nature of the waste (see CSA N292.0-14 (2014)). General principles for the management of radioactive waste and irradiated fuel, for detailed information on classification and management. There are different methods for storing each type of radioactive waste.
Low and intermediate-level wastes consist of all non-fuel waste arising from the activities associated with nuclear electricity generation, nuclear research and development, and from the production and use of radioisotopes in medicine, education, research, agriculture and industry. Low-level radioactive waste (LLRW) has radionuclide content above established clearance levels and exemption quantities, and generally limited amounts of long-lived radioactivity (CSA 2014b). Most LLRW requires limited shielding for handling. The most common forms of LLRW are contaminated equipment and materials, rags, protective clothing and contaminated soils.
Intermediate-level radioactive waste (ILRW) consists of wastes generally requiring shielding during handling and interim storage due to elevated levels of penetrating radiation. Unlike high-level wastes, ILRWs require little or no provision for heat dissipation during handling, transportation and long-term management (CSA 2014b). Some ILRW may however, have heat generation implications in the short term (e.g., refurbishment waste) because of its total radioactivity level. The most common form of ILRW is ion exchange resins and filters.
All low and intermediate level radioactive wastes produced at the Great Lakes NPPs are transported and stored on an interim basis at the Western Waste Management Facility located adjacent to the Bruce Power site on the shore of Lake Huron. Here the LLRW is sorted, stored, compacted or incinerated. After processing, the LLRW is stored in above-ground warehouses. In contrast, ILRW is not processed. Instead, it is stored in steel-lined concrete containers and set in the ground.
High-level radioactive waste (HLRW) is irradiated nuclear fuel that has been declared waste and/or any waste that generates significant heat (i.e., typically more than 2 KW per cubic metre) as a result of radioactive decay (CSA 2014b). Such waste requires significant shielding for handling and storage as well as considerations for heat dissipation and long-term isolation. HLRW most commonly involves used nuclear fuel from nuclear power plants, prototype and demonstration power reactors, and research and isotope production reactors. These high-level wastes are stored underwater in the nuclear station's used fuel bay for seven to ten years. It is then transferred to specially designed dry storage containers stored in above-ground warehouses at each of the NPPs. Used fuel from research reactors (i.e., SLOWPOKE-2s and the McMaster nuclear reactor) are shipped to Chalk River Laboratories for storage.
Interim storage waste-management facilities
There are three interim storage waste-management facilities located on the Great Lakes basin: the Western Waste Management Facility (on Lake Huron), the Pickering Waste Management Facility (on Lake Ontario) and the Darlington Waste Management Facility (on Lake Ontario). Any radioactive effluents from these facilities are generally routed to the nearby power plant's radioactive water management system, and storm water run-off is monitored through the storm-water management program. Environmental monitoring programs associated with these facilities are incorporated within the associated NPP's radiological environmental monitoring program and is a licence requirement.
The Douglas Point Waste Management Facility is located at the site of the former Douglas Point Nuclear Generating Station on the Bruce nuclear site. All internal liquids are collected and stored in holding tanks. Liquid effluent releases are a result of the external groundwater diversion system and are 10,000 times lower than the releases from a power plant. Bruce Power Development Radioactive Waste Operations Site 1 is a storage facility located on the Bruce nuclear site that stores low- and intermediate-level waste from Douglas Point Waste Management Facility and Pickering units 1 and 4. Once again, releases from these facilities are monitored as part of the site receiving environment monitoring programs.
Long-term waste-management storage facilities
Historical low-level waste-management areas: Port Hope and Clarington municipalities
The historic LLRW contamination found in Port Hope and Port Granby is a result of the area's long history of involvement with the nuclear industry. This legacy extends back to the 1930s with the commencement of radium refining activities by Eldorado Gold Mines Ltd. In the 1940s, Eldorado switched its focus to uranium processing and became a Crown corporation. During those early years of refining and processing, the need for careful management of process wastes was not well recognized. Consequently, these wastes were stockpiled at various locations throughout the community, and used for fill material in construction and landscaping.
In 1948, Eldorado Nuclear began placing process wastes in the Welcome Waste Management Facility (WMF) in Port Hope. The Welcome WMF continued to receive new waste until 1955, when Eldorado began operating the Port Granby WMF, located in nearby Clarington, Ontario. The Port Granby WMF received process wastes and other waste materials until 1988. Although these facilities represented an improvement from the initial practices of Eldorado Gold Mines Ltd., it was recognized that the WMFs did not provide for acceptable long-term management of LLRW. A solution was therefore necessary to upgrade these facilities and to remedy the LLRW contamination that had resulted from activities of the former Crown corporation. This solution became the Port Hope Area Initiative (PHAI).
The PHAI represents the Government of Canada's commitment to the development and implementation of a safe, local, long-term management solution for historic LLRW in the municipalities of Port Hope and Clarington (see figure 14). It consists of two projects, reflecting the work being done in each municipality: the Port Hope Project and the Port Granby Project.
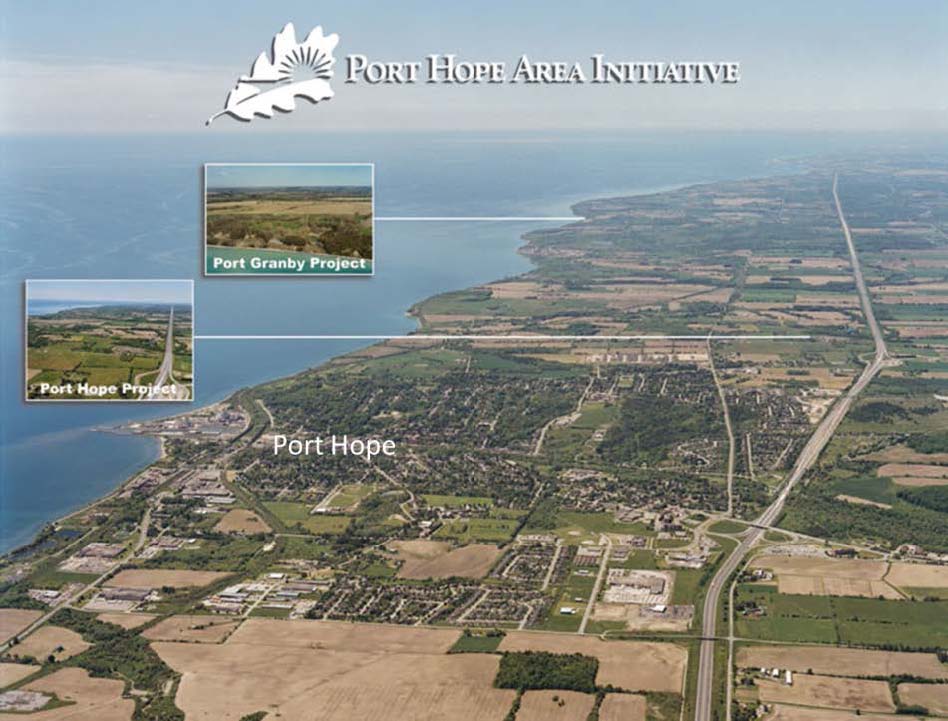
Descriptive text for Figure 14
Figure 14 shows an aerial view from the east of the Port Hope Area Initiative with the locations of the Port Hope project and Port Granby Project highlighted.
Both projects involve the relocation of waste to two separate long-term waste-management facilities consisting of multi-layered engineered mounds. Each facility is engineered with a base liner consisting of multiple layers of natural and synthetic materials to prevent the release of contaminants to the environment. Within the base liner, a leachate collection system will be installed to direct leachate for treatment. Upon placement of the LLRW on the base-liner, a multi-layered cover system will be constructed. Once complete, radiation levels on the mounds are expected to be similar to natural background levels.
Each project includes new state-of-the-art water treatment plants that were commissioned in 2015–16, and designed to meet the needs of the specific liquid wastes they will be treating. Both plants utilize multi-stage treatment processes involving initial separation of solids for disposal in the engineered mounds followed by reverse osmosis treatment. (Port Granby uses biological reactors, while Port Hope uses chemical precipitation.) The clean stream from the reverse osmosis treatment is released to Lake Ontario; the dirty stream is directed through an evaporator system with remaining solids disposed of in the engineered mounds.
Temporary improvements and increased monitoring commenced in 2011 at the old WMFs (i.e., Welcome and Port Granby) until the new treatment systems began operating in 2015–16. For both these sites, associated monitoring of Lake Ontario surface waters from 2012 to present showed annual mean and maximum concentrations of uranium and radium-226 at concentrations below drinking water guidelines and guidelines for the protection of aquatic life.
The Port Granby Project is to receive wastes relocated from the current Port Granby WMF. The Port Hope Project will receive waste relocated from the Welcome WMF plus additional materials from various remediation sites throughout Port Hope. Of significant relevance to this assessment will be the relocation of the contaminated Port Hope Harbour sediments – which are classified as an area of concern under the GLWQA – to the Port Hope long-term waste-management facility. Approximately 120,000 m3 of contaminated sediments are to be removed from the harbour. As these contaminants are removed from the Great Lakes, they will no longer measurably contribute radionuclides to the Great Lakes.
The PHAI has completed the required environmental assessments under the Canadian Environmental Assessment Act (1992) as well as those required to receive the necessary permits and licenses from the CNSC and other federal and provincial regulatory bodies to commence the final remediation. Both projects have commenced activities and completed construction of their new water treatment plants. More information on the PHAI can be obtained from their website including construction schedules and environmental monitoring programs and their results. Information on CNSC regulatory activities, licensing and the CNSC's IEMP associated with these projects are available on the CNSC's website.
These new long-term waste management systems will significantly reduce radionuclide loads (and other hazardous substances) to Lake Ontario associated with these historical wastes.
Proposed Deep Geologic Repository for low-level and intermediate waste
Ontario Power Generation is currently seeking regulatory approval for the construction and operation of a proposed Deep Geologic Repository (DGR) near Lake Huron. The DGR would store low and intermediate-level radioactive waste underground in a stable geologic environment. The Minister of Environment and Climate Change Canada has yet to make a decision on the environmental assessment of the project. If approved, this project would relocate waste currently managed on-surface to below-ground. This change would significantly lower the long-term risk of releases to the Great Lakes.
The Nuclear Waste Management Organization (NWMO) Adaptive Phase Management (APM) project seeks to find a solution for the long-term management of used nuclear fuel – a solution that is socially acceptable, technically sound, environmentally responsible and economically feasible to Canadians. The project remains in the planning stages. No site has been selected, nor has a licence application under the NSCA been received. As of June 2017, six areas remained the focus of the site-selection process. A number of those areas are located within the Great Lakes basin. Further information on the APM process can be found at the NWMO website.
3.2.6 Nuclear processing facilities and non-power research reactors
A brief introduction is provided for each of these facilities to ensure complete coverage of nuclear facilities. None of these facilities has direct releases to the Great Lakes.
Tritium processing
SRB Technologies is the only operating tritium processing facility in Canada. SRB Technologies uses tritium to make various products, such as self-luminous emergency exit signs. Its facility is located in Pembroke, Ontario and thus is not associated with the Great Lakes basin. Shield Source Incorporated, a tritium processing facility located near Peterborough, Ontario was permanently shut down in 2014.
Medical isotope processing
Chalk River Laboratories is a nuclear research facility that conducts research and development of nuclear technologies. Chalk River Laboratories operates the National Research Universal reactor. The National Research Universal reactor supports and advances the design of the CANDU, produces neutrons, and produces medical radioisotopes. Nordion is a company that also produces radioisotopes for medical and industrial applications. Neither of these facilities is located near the Great Lake basin.
McMaster Nuclear Reactor
The McMaster University research reactor is operated by McMaster University for research, education and commercial services. The McMaster Nuclear Reactor does not have direct releases to the Great Lakes or to the sewers.
SLOWPOKE-2
There are currently four operating SLOWPOKE-2 reactors. These are located at the University of Alberta, the Saskatchewan Research Council, the Polytechnique Montréal and the Royal Military College. The Royal Military College is the only SLOWPOKE-2 facility situated near a Great Lake (i.e., the St. Lawrence River outlet of Lake Ontario). The facility does not have direct releases to surface water and releases effluent to sewer only when the radioactivity is within background levels.
3.2.7 Medical applications
Radionuclides play a significant role in Canada's healthcare system for both diagnostic and therapeutic purposes. Use of these materials is strictly regulated, as is the management and disposal of wastes associated with medical practices. Only aqueous medical liquid wastes are allowed to be released to municipal sewer systems.
As medical radioisotopes are short-lived (i.e., half lives of minutes to hours), most are managed through retention in holding tanks to allow complete or partial decay prior to release. Those with slightly longer half-lives, usually in the order of days (e.g., iodine-131 has a half life of 8.5 days) are managed to ensure compliance with release criteria within a facility's licence. The release to sewer limits is based on radionuclide specific clearance levels. Disposal limits are either generic or facility specific. Radionuclide specific generic clearance levels are based on highly conservative models limiting exposure to less than 0.01 mSv/year (compared to public dose limit of 1 mSv/year) (IAEA 1998). Site-specific disposal limits are derived for situations where the assumptions associated with the generic clearance values are not considered to be adequately conservative for a facility.
As a result of these waste management practices and the short half-lives associated with these radionuclides, releases from medical facilities are not expected to adversely affect human health or the Great Lakes ecosystem.
3.2.8 Non-nuclear industrial sources
Nuclear activities are not the only activities that can raise radionuclide concentrations in surface waters. Most non-nuclear industrial activities with this potential are associated with processing materials that naturally contain elevated levels of primordial radionuclides that are commonly referred to as naturally occurring radioactive material (NORM). Thus, the dominant radionuclides are those associated with uranium and thorium decay chains. Potassium-40 is the most important non-uranium series radionuclide among them.
The main industrial activities of interest are phosphate mining and phosphate fertilizers, fossil fuel extraction and energy production and non-uraniumFootnote 4 mining mineral extraction. Regulation of these activities is a provincial responsibility in Canada with assistance provided by an intergovernmental committee known as the Federal Provincial Territorial Radiation Protection Committee. A NORM working group within the FPTRPC has produced a guideline document titled Canadian Guidelines for the Management of Naturally Occurring Radioactive Materials - NORM (HC 2011). There are also a number of IAEA documents for helping member states to regulate these activities.
Agricultural phosphate is obtained through the extraction of phosphate rock. This is often found in sedimentary rock formations interbedded with marine shales or limestones. This rock can have elevated uranium concentrations. Uranium and associated daughters are present within waste products and the fertilizer end-product. The application of phosphate fertilizers can result in an increase in soil radionuclide content as well as that of associated streams. These do not significantly affect the dose received by the general population. Depending on the radionuclide content of the ore, there can be a need for precautions to protect workers and a need to consider radiation hazards for appropriate decommissioning of a site to make it suitable for the final end-use. IAEA Safety Report Series No. 78, Radiation Protection and Management of NORM Residues in the Phosphate Industry provides detailed recommendations for managing and regulating these activities (IAEA 2013).
Canada is not identified as being among the top-10 phosphate producing regions, and the CNSC is not aware of any significant phosphate rock and fertilizer and production on the Canadian side of the Great Lakes basin.
Coal contains radionuclides of the uranium and thorium decay series and potassium-40. The extraction process and the burning of coal can slightly increase environmental concentrations fractionally above background. Coal fly ash can concentrate lead-210 and polonium-210 up to five to ten times greater than in the original coal, and thus must be appropriately managed. Modern fly ash regulations tend to address this appropriately.
The last coal electrical generating plant on the Canadian side of the Great Lakes basin was closed in 2014. In 2015, the Ontario government passed legislation permanently banning coal-fired electricity generation in the province. Thus, coal is no longer a potential source of radionuclides for the Canadian side of the Great Lakes basin.
Radionuclides associated with oil and gas production have recently received increased attention, especially with increased North American production from fracking. Radionuclides of the uranium and thorium decay series can accumulate in various components associated with the recovery process. This can lead to concentration in scales deposited in wellheads, valves, pumps, separators, water treatment vessels, gas treatment and oil storage tanks (IAEA 2003). Radionuclide content may need to be considered when handling and disposing of these materials. Production waters may also contain low levels of radionuclides requiring consideration before releasing to the environment. The level of radioactivity varies significantly, depending on the radioactivity of the reservoir rock and the salinity of the water co-produced from the well. The higher the salinity, the more NORM is likely to be mobilized.
Guidance for managing and regulating these activities can be found in IAEA Safety Report Series No. 34, Radiation Protection and the Management of Radioactive Waste in the Oil and Gas Industry (IAEA 2003). Industry best practices guides are also available (International Association of Oil and Gas Producers (IAOGP) 2008). The CNSC is not aware of any significant oil and gas recovery activities occurring on the Canadian side of the Great Lakes basin that would be considered significant contributors of radionuclides to the Great Lakes, nor would the CNSC have any regulatory authority over such activities.
The last activity to be discussed is the extraction of rare earth elements. Rare earth is often used as a generic term applied to a range of elements (e.g., lanthanoids, scandium and yttrium) more commonly extracted from mineral deposits of bastnaesite and monazite. Interest in rare earth mining is increasing due to the elements' importance in advanced high-technology products ranging from wind turbines to hybrid cars. These deposits are often associated with thorium and to a lesser extent uranium. This, combined with the fact that they are usually found in low concentrations requiring the processing of significant amounts of material, can result in the creation of low activity wastes. In some instances, uranium can actually be processed as a commercially viable byproduct as is proposed for the Kvanefjeld deposit in Greenland. This represents good environmental practice as processing the uranium as a byproduct removes it from the tailings.
In summary, there are currently no significant industrial activities involving NORM on the Canadian side of the Great Lakes basin that would result in a measurable effect on water quality or the ecology of the Great Lakes.
4.0 Generic risk assessment
Within the previous sections, radionuclide environmental monitoring results associated with the various Canadian nuclear facilities were assessed against media-specific criteria. From this information, it can be stated that radionuclides within the Great Lakes do not pose an unreasonable risk to health of people when considering single radionuclides along single-exposure pathways (e.g., drinking water and fish consumption). However, radiation exposures are integrated across multiple radionuclides and exposure pathways to provide total doses. It is the total dose that is of interest when assessing potential impacts on human health or on non-human biota. To address this issue, a conservative screening risk assessment has been completed for multiple pathways and multiple radionuclides within the Great Lakes.
For this analysis, a database was created containing the results of the radionuclide environmental monitoring programs for CNSC-regulated facilities along the Great Lakes from 2006 to 2015. This information was supplemented by data from the provincial water supply monitoring database from 2006 to 2012.
4.1 Human health risk assessment – public dose
As previously discussed in section 3.2.4, facility-specific doses to members of the public are in the range of 1–3 µSv/year, which is a small fraction of the dose resulting from natural background and less than 0.5% of the public dose limit. These assessments are site-specific, and incorporate local characteristics that may not be representative of more sensitive sub-populations within the Great Lakes basin. To address this, a bounding, generic screening assessment has been completed for a range of theoretical representative persons experiencing the highest potential exposure pathways generated from monitoring data collected throughout the Great Lakes. See appendix B for details on input parameters and modelling assumptions.
Three exposure scenarios were assessed:
- radionuclides associated with nuclear power plants
- radionuclides associated with fuel refining and conversion facilities
- a scenario combining the highest exposure values (i.e., from either Lake Huron or Lake Ontario) for both facility categories combined
In all instances, dose estimates from the main exposure pathways to Great Lakes radionuclides are extremely low (i.e., three orders of magnitude below the public dose limit) (see table 8). The main exposure pathway for NPP scenarios is drinking water associated with the slight increase in tritium from drinking water plants near NPPs. The main dose contributor for uranium refining and conversion facilities is associated with fish ingestion using conservative assumptions for transfers of radionuclides from water to fish. The most sensitive representative person tends to be Indigenous peoples due to the higher fish ingestion rates for this sub-population for all assessment scenarios.
Source of public exposure | Dose: drinking water | Dose: immersion | Dose: fish ingestion | Dose: external sediment | Dose: total (%)2 | Most sensitive representative person |
---|---|---|---|---|---|---|
Nuclear power plants | 2.8 x 10-3 | 7.6 x 10-5 | 6.7 x 10-6 | 5.9 x 10-7 | 3 x 10-3 (0.3%) | Indigenous adult |
Fuel refining and conversion | 3.3 x 10-4 | 6.6 x 10-7 | 2.7 x 10-3 | 1.2 x 10-3 | 4 x 10-3 (0.4%) | Indigenous infant |
All facilities combined | 2.7 x 10-3 | 1.0 x 10-4 | 2.7 x 10-3 | 1.2 x 10-3 | 7 x 10-3 (0.7%) | Indigenous child |
1 see appendix B for calculations 2 Total dose as a percent of the public dose limit of 1 mSv/year. Public dose limit is 1 mSv/year. |
These extremely low doses, despite the conservative assumptions, indicate that further sampling and analyses of additional radionuclides and exposure pathways beyond the more detailed site-specific monitoring and dose calculations already completed for licensing purposes, although of scientific interest, are not justified from a risk assessment and management perspective.
4.2 Ecological risk assessment
In 2004, a comprehensive ecological risk assessment (EC and HC 2004) of the Canadian nuclear fuel cycle was released under the Canadian Environmental Protection Act 1999 (CEPA 1999). The assessment concluded the following:
"Based on available data concerning the effects from exposure to ionizing radiation, it has been concluded that ionizing radiation emitted by radionuclides released from uranium mines and mills, uranium refineries and conversion facilities, stand-alone waste management facilities, power reactors and their associated waste management facilities, and research reactors is not (emphasis added) entering the environment in quantities or concentrations or under conditions that have or may have an immediate or long-term harmful effect on the environment or its biological diversity." (EC and HC 2004Footnote 5).
Thus, from an ionizing radiation perspective, radionuclides associated with the Canadian nuclear fuel cycle were not considered to be CEPA toxic to non-human biota and posed no unreasonable risks to the environment. The assessment did conclude that uranium as a chemical substance (e.g., acting as a heavy metal) released from uranium mines and mills was CEPA toxic based on three sites in northern Saskatchewan. Releases of uranium at the identified facilities have been addressed and procedures are in place for other facilities to ensure uranium does not pose a problem at any future sitesFootnote 6. Moreover, these sites are located in northern Saskatchewan and do not pose a risk to the Great Lakes basin.
Since the CEPA assessment, a series of models for calculating doses to non-human biota, a transfer factor handbook and proposed exposure benchmarks have been published in the scientific literature and undergone evaluation by national and international regulatory bodies. The New York State Department of Environmental Conservation provides a review of these aspects in a recent assessment report (NY DEC 2014). Two of these models, the U.S. DOE RESRAD-BIOTA and the European Union ERICA Tool are utilized here to evaluate radionuclide data specific to the Great Lakes in section 4.2.1 and 4.2.2. Additional details on the calculations are provided in appendix C.
4.2.1 Nuclear power plants on the Great Lakes
Following Brown et al. (2003), concentrations of radionuclides in water, sediment and fish tissue from exposure associated with nuclear facilities and reference locations were converted into a dose rate using the ERICA Tool (Brown et al. 2016) and RESRAD-BIOTA (DOE 2004) models. Doses derived from these models were compared to the UNSCEAR aquatic screening benchmark of 400 μGy/h (approximately 10 mGy/day) by RESRAD-BIOTAFootnote 7 and the significantly more stringent generic screening value of 10 µGy/h (Andersson et al. 2009) used by the ERICA Tool (Brown et al. 2016).
A radionuclide dose assessment using the ERICA Tool (Brown et al. 2008, 2016) was completed for benthic and pelagic fish, using the most conservative model parameters. An extremely conservative approach (i.e., over-predictive) was taken for assessing dose to non-human biota from sediment exposure. Modelling of carbon-14 exposure to the sediments requires correction for organic content of the sediments. The highest recorded organic carbon content for sediments from a recent sediment Great Lakes survey was selected (OPG 2014) for this purpose.
The significantly more conservative default screening dose rate of 10 µGy/h contained in the ERICA model is based on species sensitivity distribution derived from the scientific literature. This is considered to represent a chronic no-effects-level dose rate below which one would expect no effects on populations of aquatic biota (Garnier-Laplace and Gilbin 2006, Garnier-Laplace et al. 2008).
Model results, shown in table 9, demonstrate that potential dose rates near the Bruce, Pickering, and Darlington NPPs would be lower than the ERICA dose threshold of 10 µGy/h (Brown et al. 2008) and substantially below UNSCEAR threshold of 400 µGy/h for fish. This holds true even with the conservative assumptions inherent in the ERICA Tool and those applied for carbon-14 and carbon content for sediment exposure. In addition, the estimated dose rates are similar between those calculated for reference areas and those located in proximity to the NPPs showing that radiological effluent releases are having a negligible impact on aquatic biota.
Site | Exposure (µGy/h) | Reference (µGy/h) |
---|---|---|
Radionuclides in water (tritium and gross beta) | ||
Benthic fish | ||
Bruce | 5.09 x 10-2 | 4.50 x 10-2 |
Pickering | 6.19 x 10-2 | 4.50 x 10-2 |
Darlington | 7.32 x 10-2 | 4.50 x 10-2 |
Pelagic fish | ||
Bruce | 4.90 x 10-2 | 4.34 x 10-2 |
Pickering | 5.97 x 10-2 | 4.34 x 10-2 |
Darlington | 7.05 x 10-2 | 4.34 x 10-2 |
Radionuclides in sediment (cesium-137, carbon-14, cesium-134 and cobalt-60) | ||
Benthic fish | ||
Bruce | 7.68 | 5.76 |
Pickering | 7.68 | 5.76 |
Darlington | 4.99 | 5.76 |
Pelagic fish | ||
Bruce | 7.68 | 5.76 |
Pickering | 7.68 | 5.76 |
Darlington | 4.99 | 5.76 |
Therefore, these results indicate that the fish populations in close proximity to nuclear power plants are not likely to be affected by the levels of radionuclides in the water and sediments and that less conservative more realistic modelling is not warranted.
Additional model runs were made applying the U.S. Department of Energy's graded approach (DOE 2002) with conservative parameters for aquatic and riparian species exposed through a suite of radionuclides in Great Lakes water and sediment. The RESRAD default screening dose rate of 10 mGy/day (∼ 416 µGy/h) was applied.
Results of this modeling exercise, presented in table 10, demonstrate that the average dose rate to aquatic and riparian species is comparable to the average dose rate for reference areas (background), and in both cases, results were below the screening threshold for aquatic biota.
Site | Exposure (mGy/day) | Reference (mGy/day) |
---|---|---|
Radionuclides in water (tritium and gross beta) | ||
Aquatic animal | ||
Bruce | 4.74 x 10-4 | 4.20 x 10-4 |
Pickering | 5.77 x 10-4 | 4.19 x 10-4 |
Darlington | 6.82 x 10-4 | 4.19 x 10-4 |
Riparian animal | ||
Bruce | 8.85 x 10-3 | 7.87 x 10-3 |
Pickering | 1.08 x 10-2 | 7.87 x 10-3 |
Darlington | 1.28 x 10-2 | 7.87 x 10-3 |
Radionuclides in sediment (cesium-137, carbon-14, cesium-134 and cobalt-60) | ||
Aquatic animal | ||
Bruce | 6.58 x 10-1 | 4.93 x 10-1 |
Pickering | 6.58 x 10-1 | 4.93 x 10-1 |
Darlington | 4.27 x 10-1 | 4.93 x 10-1 |
Riparian animal | ||
Bruce | 8.52 x 10-1 | 6.39 x 10-1 |
Pickering | 8.52 x 10-1 | 6.39 x 10-1 |
Darlington | 5.53 x 10-1 | 6.39 x 10-1 |
Therefore, it can be concluded that radionuclides associated with nuclear power plant operations in the Great Lakes contribute a negligible radiation dose to non-human biota and do not pose a risk to the aquatic environment.
These screening assessments using the latest dose models (i.e., the RESRAD-BIOTA and ERICA Tool) support the conclusions of the previous CEPA toxic assessment for radionuclides. Radionuclides associated with releases from NPPs are not resulting in exposures to ionizing radiation at levels that would affect non-human biota.
4.2.2 Nuclear processing and waste-management facilities on the Great Lakes
A radionuclide dose assessment for aquatic and riparian animals was done using RESRAD-BIOTA using conservative parameters (DOE 2004), similar to those used for the previous assessment on NPP releases. The calculated absorbed doses for modelled aquatic and riparian wildlife exposed to the measured radionuclides concentrations in the water and sediments near nuclear processing and waste management facilities are provided in table 11. Absorbed doses for all scenarios are at least two orders of magnitude below the screening dose rate criterion of 10 mGy/d indicating populations of non-human biota are not likely to be affected. Doses between reference and exposure populations are similar with the exception of the PHCF. These higher doses are primarily a result of exposure to the uranium series radionuclides in the harbour sediments. As previously mentioned, these sediments have already been identified under the GLWQA as an area of concern. They are being removed as part of the PHAI and will no longer be a source of exposure in the future.
Site | Aquatic animal (mGy/d) | Riparian animal (mGy/d) | ||
---|---|---|---|---|
Exposure | Reference | Exposure | Reference | |
Port Hope Conversion Facility (Lake Ontario) | 4.93 x 10-1 | 2.71 x 10-2 | 1.47 x 10-2 | 8.09 x 10-4 |
Port Granby WMF (Lake Ontario) | 2.44 x 10-1 | 2.14 x 10-1 | 7.54 x 10-2 | 6.35 x 10-2 |
Blind River Refinery (Lake Huron) | 1.69 x 10-1 | 1.93 x 10-1 | 4.01 x 10-2 | 4.23 x 10-2 |
Similarly, a radionuclide dose assessment for benthic and pelagic fish was completed using the ERICA Tool (Brown et al. 2016), applying the model's most conservative parameters and the more stringent ERICA generic screening value of 10 µGy/h. Results from this modeling exercise are presented in table 12. Findings are similar to those of the RESRAD analyses. All doses are an order of magnitude below the ERICA screening threshold indicating there is little risk to benthic and pelagic fish species. Again, there is little difference between absorbed doses for the exposure and reference scenarios with the exception of the PHCF scenario. As discussed this is a result of the contaminated harbour sediments scheduled for remediation. However, even with this known contamination, there is little radiological risk to fish.
Site | Benthic fish (µGy/h) | Pelagic fish (µGy/h) | ||
---|---|---|---|---|
Exposure | Reference | Exposure | Reference | |
Port Hope Conversion Facility (Lake Ontario) | 7.28 x 10-1 | 4.01 x 10-2 | 7.28 x 10-1 | 4.01 x 10-2 |
Port Granby WMF (Lake Ontario) | 3.50 x 10-1 | 5.71 x 10-1 | 3.11 x 10-1 | 5.00 x 10-1 |
Blind River Refinery (Lake Huron) | 2.08 x 10-1 | 2.42 x 10-1 | 1.69 x 10-1 | 2.02 x 10-1 |
The modelled doses to aquatic and riparian biota using the latest versions of RESRAD and ERICA continue to support the conclusions of the previous CEPA toxic assessment (EC and HC 2004) that ionizing radiation associated with releases of nuclear facilities impart a negligible dose to non-human biota and thus are not posing an unreasonable risk to the environment.
Part I conclusion
Current health and environmental science clearly indicates that radionuclides may pose a risk to both human and ecological health if not properly managed and regulated. As a result, an international and national radiation protection framework has been established based on the latest science and best management practices. Standards and risk assessment methodology and benchmarks have been derived from the latest science for the protection of human health and the environment.
There is currently a reasonable understanding of concentrations of the key radionuclides within the Great Lakes basin as a whole and a very good dataset and knowledge of radionuclide concentrations in close proximity to major nuclear facilities. The primary conclusion from the available data is that the most significant contribution to radionuclides within the Great Lakes basin as a whole has been fallout from atmospheric nuclear weapons testing. As a result, current radiation levels in the mixed waters of the Great Lakes area are actually significantly lower than they were in the 1960s. Thus, despite increased nuclear activity since the 1970s, radionuclide exposure as a whole to populations in the Great Lakes basin has actually decreased with the decay of fallout radionuclides.
After fallout, tritium associated with CANDU nuclear power reactors becomes the primary radionuclide of interest. For this reason, tritium has received a substantial amount of attention with respect to research and monitoring (see section 5.3). Great Lakes tritium concentrations are modelled and periodically field verified to support the public dose assessments required of Canadian NPPs. Tritium, along with gross alpha and beta are also extensively monitored at water supply plants along the Canadian shores of the Great Lakes (see section 3.2.4).
Extensive monitoring data are available for radiological releases from nuclear facilities as well as for the local
environment associated with these facilities. Radionuclides of secondary interest are also monitored as part of
these facility-specific programs.
Based on the extremely well developed radiation health sciences, there is no evidence to indicate that radionuclides
currently within the Great Lakes are posing an unreasonable risk to the environment or to the health and safety of
persons.
Part II: Current management and regulatory practices
5.0 International and national science and regulatory frameworks
There currently exists an extremely robust science and regulatory network both internationally and nationally for radionuclides.
5.1 International science and radiation safety framework
As discussed in section 2.3, there exists an international network of scientific and regulatory bodies focusing on the protection of humans and the environment from exposure to radiation and radionuclides (see figure 3). The scientific basis is provided by UNSCEAR. The science from UNSCEAR feeds directly into the activities of the ICRP, which uses this information to maintain and expand as necessary the ISRP. This system serves worldwide as the basis for radiological protection standards, legislation, guidelines, programs and practices to control industrial and medical applications of nuclear technology and materials. Using the science of UNSCEAR and the radiation protection system of the ICRP, the IAEA develops safety standards and protection programs to assist member states in their management and regulatory oversight of nuclear activities.
An additional important service of the IAEA is the Integrated Regulatory Review Service (IRRS). The IRRS is designed to strengthen and enhance the effectiveness of the national regulatory infrastructure of states for nuclear, radiation, radioactive waste and transport safety. IRRS missions are focused audits of member states' regulatory technical and policy frameworks against international guidelines embodied in the IAEA Safety Standards and of good practices observed in other member states. The CNSC participates in the IRRS both as evaluation team members and as the subject of IRRS reviews.
The CNSC incorporates the information from this international network to ensure that Canada's regulatory framework remains current and consistent with the best science. An example of this is the proposed amendments to the Radiation Protection Regulations to incorporate the latest UNSCEAR and ICRP recommendations.
5.2 Regulation of radioactive materials and nuclear activities in Canada
Formal regulation of nuclear activities in Canada commenced in 1946 with the coming in to force of the Atomic Energy Control Act and the resultant creation of the Atomic Energy Control Board.
Subsequently, the Nuclear Safety and Control Act (NSCA) came into force in May 2000, replacing the Atomic Energy Control Act. The NSCA reaffirmed the federal government authority over the regulation of activities associated with the nuclear fuel cycle in Canada and represented a modernization of Canada's nuclear regulatory regime.
The specific objectives under the NSCA are to:
-
regulate the development, production and use of nuclear energy and the production, possession and use of nuclear
substances, prescribed equipment and prescribed information in order to:
- prevent unreasonable risk to the environment and to the health and safety of persons, associated with that development, production, possession or use
- prevent unreasonable risk to national security associated with that development, production, possession or use
- achieve conformity with measures of control and international obligations to which Canada has agreed
- disseminate objective scientific, technical and regulatory information to the public concerning the activities of the Commission and the effects, on the environment and on the health and safety of persons, of the development, production, possession and use
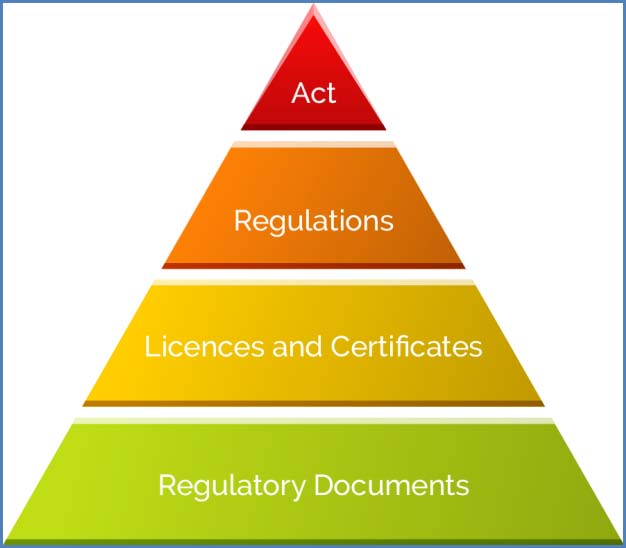
Descriptive text for Figure 15
Figure 15 is a visualization of the hierarchical structure of the CNSC regulatory framework. The figure is a pyramid with acts at the top and, in descending order: regulations, licenses and certificates, and regulatory documents
The CNSC can be broadly separated into two parts: a decision-making body consisting of the Commission (up to seven permanent members) and the Commission staff responsible for ensuring the implementation of Commission's decisions, the management and maintenance of the NSCA and regulations, and the day-to-day operation of the organization. The Commission is an independent administrative tribunal set up at arm's length from government, with ties to neither the nuclear industry nor to any specific federal minister. The Commission reports directly to Parliament. The Commission has all the powers necessary to carry out its duties with respect to the appearance, summoning and examination of witnesses, the production and inspection of records, the enforcement of its orders, and any other matter necessary for the due exercise of its jurisdiction.
The CNSC's regulatory framework (see figure 15) consists of laws passed by Parliament that govern the regulation of Canada's nuclear industry, and regulations, licences and documents that the CNSC uses to regulate the industry. The CNSC is committed to providing regulatory instruments that make its expectations clear. Regulatory documents are a key part of the CNSC's regulatory framework for nuclear activities in Canada. They explain to licensees and applicants what they must achieve to meet the requirements set out in the NSCA and the associated regulations. Regulatory documents may also contain practical guidance and suggestions to licensees and applicants on how to meet the CNSC's regulatory requirements.
Assuring compliance with legislation, regulations and licensing requirements is one of the CNSC's core business processes and is carried out through compliance verification and enforcement. Compliance verification includes site inspections and the review of operational activities and licensee documentation. CNSC inspectors are designated and empowered under the NSCA to enforce regulatory requirements.
The CNSC uses a graduated approach to enforcement to encourage and compel compliance and deter future non-compliances. When a non-compliance (or a continued non-compliance) has been identified, CNSC staff assess the significance of the non-compliance, and determine the appropriate enforcement action, based on the CNSC's graduated approach to enforcement.
The Commission makes decisions on the licensing of major nuclear facilities through a public hearing process. The public hearing gives involved parties, members of the public and Indigenous groups an opportunity to be heard before the Commission. Following a public hearing, the Commission deliberates and makes its decision. The Commission also holds public Commission meetings. Commission proceedings are webcast and available for viewing by interested parties. To help facilitate public and Indigenous participation in CNSC-related matters, a participant funding program is in place.
The CNSC ensures reporting transparency by making all annual compliance reports and regulatory oversight reports available to the public. This includes publicly posting the results of national and international (e.g., IRRS) audits and CNSC responses to those audits.
5.2.1 Protection of the environment under the NSCA
The CNSC, under the NSCA, is responsible for ensuring that licensed nuclear facilities are operating in a safe manner that ensures the protection of the environment and the health and safety of people. REGDOC-2.9.1, Environmental Protection: Environmental Principals, Assessments and Protection Measures documents the CNSC framework and expectations for this mandate area. In accordance with the NSCA and its regulations, and as a requirement of their licences, each licensee is required to develop and maintain an environmental protection program addressing all aspects of its facility or activity with the potential to influence the environment.
The environmental protection program consists of an environmental policy with commitments to the application of ALARA (for radionuclides) and best available technology economically achievable (BATEA) (for hazardous substances), the "polluter pays" and precautionary principles, and the concepts of pollution prevention, sustainable development and adaptive management.
The core elements to an environmental protection program for a major facility will include an:
- environmental risk assessment
- emissions and effluent monitoring program
- environmental monitoring program
- environmental management system
The relationships among these elements are shown in figure 16 along with the corresponding CSA standards addressing the requirements for each element. The process commences with an environmental assessment completed under either the Canadian Environmental Assessment Act, 2012 or the NSCA. An element of this assessment involves the completion of an environmental risk assessment (ERA) consisting of an ecological risk assessment and a human health risk assessment.
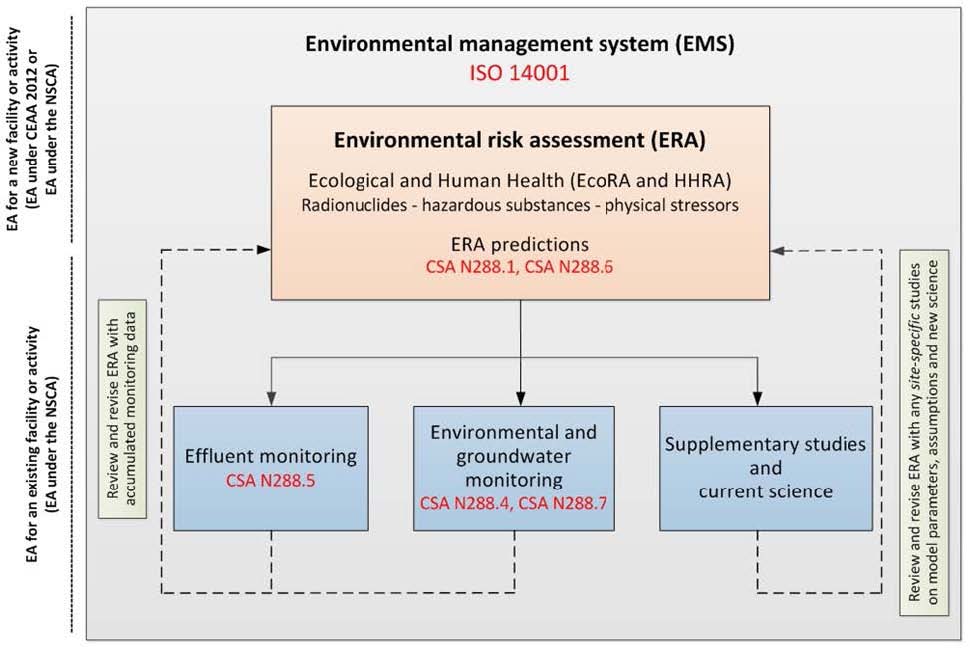
Descriptive text for Figure 16
Figure 16: A box schematic which represents the inter-relationship between the core environmental protection measures and their associated CSA standards, required of major nuclear facilities regulated by the CNSC. The core element is the environmental risk assessment (ERA) which is used to design the routine effluent, receiving environment and groundwater monitoring programs as well as any necessary special studies or investigations. On a periodic basis (no longer than 5 years) the results of the monitoring program and any special studies are to be used to revise the facilities ERA to assess performance against predictions and determine whether future performance continues to be as expected. The revised ERA is then used to revise the monitoring programs as necessary. All of these environmental protection measures or programs are to be managed within the facilities environmental management system.
The CNSC ensures that licensees have effective control measures (e.g., wastewater treatment systems, air pollution control technologies, engineered and administrative barriers and other techniques) in place to prevent or minimize releases to the environment. These preventative and control measures are expected to implement technologies and techniques that would be considered ALARA and BATEA. Release limits are established within the licence along with regulatory action levels. Action levels, set well below licence limits, act as an early warning system to ensure that licensees are carefully monitoring their operation and performance, and to ensure release limits are not reached.
The effluent monitoring program serves to measure the releases of radiological and hazardous substances in air and water to the environment and to ensure releases are below licence release limits. In addition, this program is required to address any additional radiological or hazardous substances identified on a site-specific basis through the ERA that merit monitoring.
An environmental monitoring program is used to measure the concentrations of nuclear and hazardous substances in different environmental media (e.g., air, water, vegetation, foodstuffs and soil) to demonstrate that abiotic and biotic components of the environment and members of the public are protected. The specifics of this monitoring program are determined by regulatory requirements and the results of the site-specific ERA.
The ERA is reviewed and updated periodically (i.e., five years or earlier) with a corresponding re-evaluation of the associated monitoring programs. Revisions to the ERA are informed by the accumulated site knowledge derived from operational experience, monitoring, special investigations, and the incorporation of advances in other knowledge (e.g., scientific). All of these elements are managed within a licensee's environmental management system.
In recognition of the fact that protection of the environment in Canada is a shared federal and provincial responsibility, the CNSC cooperates with other jurisdictions and federal departments to protect the environment. Where appropriate, the CNSC may enter into formal arrangements to increase the effectiveness of environmental protection. For example, the CNSC holds memoranda of understanding with other federal departments (e.g., Fisheries and Oceans Canada, ECCC and HC).
5.2.2 The CNSC Independent Environmental Monitoring Program
In addition to requiring licensees to have comprehensive environmental monitoring programs, the CNSC itself conducts independent monitoring around major nuclear facilities. The CNSC's Independent Environmental Monitoring Program (IEMP) is used to verify that the public and the environment around licensed nuclear facilities are safe.
The IEMP is implemented for facilities in all sectors of the nuclear fuel cycle. Site-specific sampling plans are developed for each facility. These focus on measuring concentrations of contaminants in the environment at publicly accessible locations such as parks, residential communities and beaches, and in areas of interest identified in ERAs. Samples may be taken for air, water, soil, sediment, vegetation such as grass and weeds, and some food, such as fish, meat and produce.
Samples are analyzed at the CNSC's laboratory for both radiological and non-radiological contaminants related to the activities of the nuclear facility and as identified in the site-specific ERA. Contaminant levels are compared to applicable values (e.g., guidelines and natural background levels) to confirm there is no impact on human health or the environment. IEMP reports, data and interactive sampling maps are provided on CNSC's website. The IEMP and the licensee monitoring programs continue to demonstrate that the public and the environment are protected.
5.2.3 Transportation under the NSCA
In Canada, the responsibility for ensuring safe transport of radioactive materials is shared between the CNSC and
Transport Canada.
There are primarily two regulations that apply to the transport of radioactive materials: Transport Canada's
Transportation of Dangerous Goods (TDG) Regulations and the CNSC's
Packaging and Transport of Nuclear Substances Regulations, 2015 (PTNSR 2015). The purpose of both sets of
regulations is to ensure health, safety and security of the public and the protection of the environment as it
relates to the transport of radioactive material. Both the TDG and PTNSR 2015 apply to all persons who handle, offer
for transport, transport or receive radioactive materials.
In Canada, as in the case in most industrialized countries, IAEA's Regulations for the Safe Transport of Radioactive Material, 2012 Edition are used as the basis to regulate the packaging and transport of radioactive material. Through the PTNSR 2015, the CNSC regulates all aspects of the packaging of radioactive material, including the design, production, use, inspection, maintenance and repair of packages. The CNSC also regulates all phases of transport from the preparation of packages for shipment to unloading at the final destination.
The basic philosophy that has guided the development of the regulations is that safety relies heavily on the design of the transport package. Package designs are combined with additional regulatory administrative controls including labeling, placarding, documentation, quality assurance and maintenance records, and allow for radioactive material to be carried safely in all modes of transport.
All radioactive materials are transported in packages that are selected based on the nature, form and quantity or activity of the substance. There are general design requirements that apply to all package types to ensure they can be handled safely and easily, secured properly, and can withstand routine conditions of transport.
Packages designed for the transport of high-risk levels of radioactive material require certification by the CNSC before they can be used in Canada. Persons must register their use of the package with the CNSC and acknowledge that they have the necessary training to properly prepare the package for shipment. These packages are required to undergo stringent testing since improper handling of their contents can give rise to severe consequences. Testing must simulate both normal and hypothetical conditions of transport and can include free-drop testing, puncture testing, thermal testing and simulated aircraft accidents.
Pursuant to the PTNSR 2015 and the TDG regulations, consignors must have measures in place to respond in the event of an emergency involving the transport of their radioactive material. In addition, the TDG regulations require the shipper to display a 24-hour telephone number on the shipping document that accompanies a shipment of dangerous goods. The purpose of these requirements is to ensure that appropriate technical assistance is available to initial emergency responders immediately.
The PTNSR 2015 requires that all incidents be reported to the CNSC immediately. Once notified of a transport incident involving radioactive material, CNSC staff will follow up to provide appropriate technical information and advice to responders on site. CNSC staff can be deployed immediately, if needed, to assist in managing the incident.
Under the TDG regulations, the transport of certain radioactive materials also requires an emergency response assistance plan (ERAP) be developed by the consignor and approved by Transport Canada before they can be transported. An ERAP describes what is to be done in the event of a transportation accident involving certain dangerous goods. The plan is intended to assist local emergency responders by providing them with technical experts and specially trained and equipped emergency response personnel at the scene of an incident. The ERAP will describe the specialized response capabilities, equipment and procedures that will be used to support a response to incidents involving dangerous goods.
The overall safety record of the transport of radioactive material in Canada and throughout the world has historically been excellent. There have never been any serious injuries, fatalities or environmental consequences attributable to the radioactive nature of material being transported since the IAEA transport regulations were established more than 60 years ago.
5.2.4 Minimizing the risk from nuclear accidents
CELA's nomination letter (CELA 2016) highlighted concerns regarding potential releases of radionuclides to the Great Lakes from accidents, including those related to transportation.
Each nuclear power plant in Canada has multiple, robust safety systems that are designed to prevent accidents, and reduce their effects should they occur. All of these systems are maintained and inspected regularly, and upgraded when necessary, to ensure plants meet or exceed strict safety standards established by the CNSC.
Shortly after the accident at Fukushima, the CNSC launched a review of all major nuclear facilities in Canada. The
review, led by a CNSC task force, confirmed that nuclear facilities in Canada are able to withstand and respond to
credible external events such as earthquakes.
In response to the task force's recommendations and following extensive consultation activities, the CNSC
established an action plan to further strengthen the safety of nuclear power plants and other major nuclear
facilities.
The action plan was implemented in three phases (i.e., short, medium and long term) based on management direction and public consultation. The final actions were completed in 2016.
Post-Fukushima, the CNSC's emphasis shifted to prevention and mitigation taking into account defense in depth. These continuous safety improvements have resulted in enhanced accident prevention, improved consequence mitigation and increased public protection.
The potential consequences of accidents are also considerations under the NSCA as well as the Canadian Environmental Assessment Act, 2012. For example, the CNSC requires proponents to provide information on malfunctions and accidents in their technical studies (i.e., environmental impact statements) to support the conduct of environmental assessments under the Canadian Environmental Assessment Act, 2012.
For some previous environmental assessments, accidental releases directly to the Great Lakes were assessed in both the Pickering B Refurbishment and Continued Operations EA (OPG 2007) and Bruce A Refurbishment for Life Extension and Continued Operations Project (Bruce Power 2006). For both environmental assessments, the conclusions were no significant environmental effects.
CELA's nomination letter (CELA 2016) also highlighted two transportation-related activities for which there has been extensive accident analysis undertaken under the NSCA.
With respect to the transport of steam generators, CNSC staff assessed the protection of drinking water supplies as a bounding environmental and human health scenario for an accident during the loading and marine transport of Bruce Power steam generators (CNSC 2010). The assessment concluded that drinking water supplies would be protected should an accident occur.
With respect to the transport of highly enriched uranyl nitrate liquid, CNSC staff completed an assessment (CNSC 2014) that evaluated potential impacts on major water bodies during an accidental release. One scenario in this assessment involved a bridge crossing near the outlet of Lake Ontario along the Ottawa River. Given the low expected doses (i.e., a fraction of the 1 mSv public dose limit), the nature of any potential release and timely remediation efforts, no adverse effects on human health were expected.
In summary, malfunctions and accidents are thoroughly considered under the Canadian regulatory framework to ensure that risks are minimized throughout the lifecycle of a facility.
5.3 Government coordination, monitoring initiatives and research
There are various government initiatives and research activities associated with either radionuclides within the Great Lakes or radionuclides that may be, or are, released to the Great Lakes.
5.3.1 Federal Provincial Territorial Radiation Protection Committee
The Federal Provincial Territorial Radiation Protection Committee is an intergovernmental committee established to support federal, provincial and territorial radiation protection agencies in their respective mandates by:
- providing a national focus for government radiation protection agencies
- promoting the harmonization of radiation health and safety programs
- identifying emerging issues in radiation protection and recommending actions to the appropriate jurisdictions
- developing and harmonizing radiation protection standards, guidelines and input for legislation
- providing a forum for representatives of the provinces and territories, the CNSC, the Department of National Defence, HC and other federal departments and agencies
- considering requests from other governmental committees and agencies concerned with health, safety and environmental issues and liaising regularly with such committees and agencies
Activities of relevance to this assessment have been the preparation of the technical support document for the Canadian radiological drinking water guidelines (HC 2009), guidelines for assessing and managing NORM (HC 20011), intervention guidelines for managing nuclear emergencies (HC 2003) and guidelines for restricting radioactively contaminated food and water following an emergency (HC 2001).
5.3.2 Ontario reactor and drinking water surveillance programs
The Government of Ontario's Radiation Protection Monitoring Service operates a radionuclide laboratory and associated radiation monitoring equipment throughout the province under the auspices of the Ontario Ministry of Labour. The service manages the Ontario Reactor Surveillance Program, the network of which continuously monitors the environment around the province's nuclear installations. The Radiation Protection Monitoring Service also provides laboratory services in support of Ontario's Provincial Nuclear Emergency Response Plan.
A network of environmental monitoring stations has been established around Ontario's three NPPs at Bruce, Darlington and Pickering, and in the vicinity of the Chalk River Nuclear Laboratories located north of Ottawa. An additional station is in the Amherstburg area to monitor the Fermi 2 NPP south of Detroit, Michigan.
Environmental samples collected at these locations are analyzed by the Radiation Protection Monitoring Service for select radionuclides. Air, drinking water and foodstuff (i.e., milk) are also routinely sampled at all locations. The program is designed to detect radionuclide concentrations in the environment that could expose members of the public to an annual dose commitment of 0.1 mSv to a member of the public from either inhalation or ingestion. Reports are published on the Ontario Ministry of Labour's website.
The Ontario Ministry of Environment and Climate Change manages the Ontario Drinking Water Surveillance Program. This program monitors water quality at selected municipal drinking water systems for scientific and research purposes. This program is a voluntary partnership that complements the regulatory monitoring that must be completed by the operators of the drinking water systems. This program addresses a range of inorganic, organic and radiological parameters. The radiological parameters include gross alpha and beta and tritium. The results of this program are used in support of the assessment of the near-field monitoring programs completed at CNSC-regulated facilities. Downloadable data sets are available on the Government of Ontario open data website.
5.3.3 Health Canada radiation monitoring networks
HC manages a national radiation surveillance program, the Canadian Radiological Monitoring Network (CRMN), involving a series of interrelated radiation surveillance monitoring networks.
CRMN stations are located in major population centres and at the major nuclear facilities in Canada. Currently, HC and the Ontario government have coordinated activities such that the Ontario Reactor Surveillance Program addresses Ontario facilities and HC manages the monitoring stations for the Gentilly-2 Nuclear Generating Station in Quebec and the Point Lepreau Generating Station in New Brunswick. The CRMN routinely collects drinking water, precipitation, atmospheric water vapour, air particulate, external gamma dose and milk samples for radioactivity analysis.
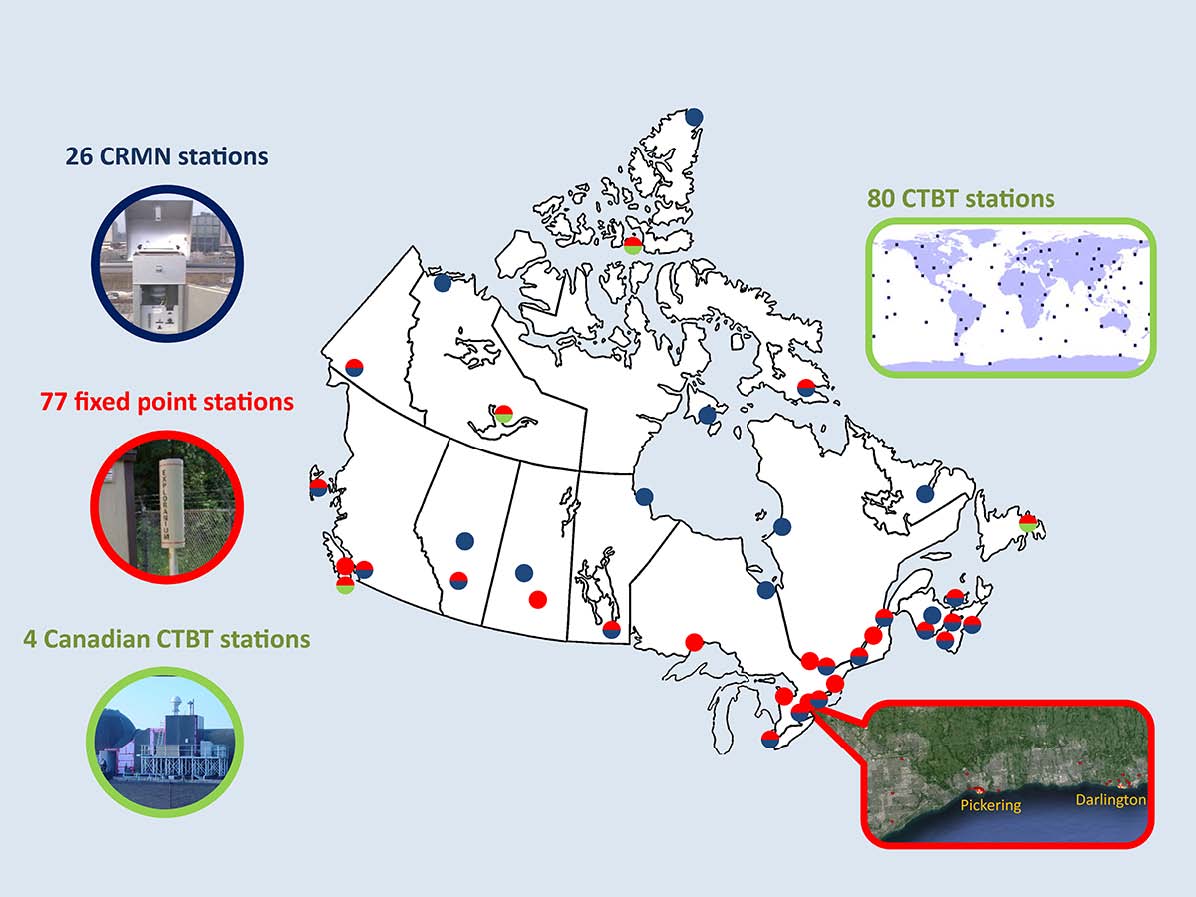
Descriptive text for Figure 17
Figure 17 shows a map of Canada with the locations of Health Canada radiation surveillance monitoring networks, the majority of monitoring stations are located along Lake Ontario, and in New Brunswick and Nova Scotia, however monitoring stations are present in every province and territory, close to coasts or population centres. Overall, across Canada there are 26 Canadian Radiological Monitoring Network stations, 77 fixed point stations, and 4 Comprehensive Nuclear-Test Ban Treaty stations; this is complimentary to the 80 Comprehensive Nuclear-Test Ban Treaty stations located around the world, the locations of which are included in an inset map in this figure.
In 2000, HC complemented the CRMN network with the Fixed Point Surveillance (FPS) system. The FPS functions as a real-time radiation detection system monitoring public doses from radioactive materials in the air. It monitors atmospheric releases associated with nuclear facilities and activities both nationally and internationally. The system consists of 77 remotely operated monitoring stations across the country covering major population centres, nuclear facilities and potential berthing areas for nuclear powered vessels. These stations continuously measure gamma radiation levels from ground-deposited and airborne contaminants. From this data, external dose estimates are calculated. This information is published on the Health Canada website.
In addition to the CRMN and FPS networks, HC also manages four of the 80 stations located worldwide for measuring aerosol particulate radionuclides and radioactive noble gases in support of the Comprehensive Nuclear-Test Ban Treaty (CTBT).
The various HC radiation surveillance monitoring networks are shown in figure 17. This network is capable of detecting minor increases in radionuclide activity levels and tracing the originating source on a global (e.g., North Korean underground bomb testing) or local scale (e.g., Canadian nuclear facilities). The system is sensitive enough to detect fluctuations in releases from Canadian NPPs well within their regulatory licence limits.
5.3.4 The CNSC's research and development program
The CNSC funds a research program to obtain knowledge and information necessary to support its regulatory mission. The program provides the CNSC with access to independent advice, expertise, experience, information and other resources via contracts, grants and contributions placed in the private sector, and with other agencies and organizations in Canada and elsewhere.
Tritium has received a great deal of attention through this research program due to its importance within the Canadian nuclear fuel cycle. A significant focus of this program has involved research on the biological effects of tritium as well as the physical, chemical and biological aspects influencing tritium transport through the environment. This research has focused on tritium industrial management practices, tritium monitoring technologies, laboratory studies of tritium carcinogenicity and dosimetry, and the environmental science necessary to effectively model the environmental fate and transport of tritium and associated dose calculations. The CNSC publishes an annual report on its research and other science activities titled, The Science of Safety. This report provides information on the CNSC's Research and Support Program. The annual research report and additional scientific information on radiation and nuclear safety are available on the CNSC website.
5.3.5 Federal Nuclear Science and Technology Program
The Government of Canada has established the Federal Nuclear Science and Technology Program (FNSTP) to ensure that Canadian nuclear priorities, especially those associated with ensuring health and safety, are supported by the science and technology necessary for Canadians to benefit from nuclear technology in a safe manner. The program, managed by Atomic Energy Canada Limited supports research and technology development at the Canadian Nuclear Laboratories in Chalk River, Ontario.
Research funded through the FNSTP is sub-divided into five themes, four of which focus on health and safety. Advisory groups consisting of representatives from government agencies responsible for regulating or working within a theme area provide direction with respect to beneficial research directions.
The health theme area focuses on research supporting the development of biological applications and understanding the implications of radiation on living organisms. Within this theme, a number of research projects are investigating low-dose effects of chronic gamma or tritium beta irradiation at both the cellular and whole animal levels of biological organization.
The environment theme area focuses research on environmental stewardship and radioactive waste management. A significant amount of the research in this theme area is of indirect and direct relevance to radionuclides within the Great Lakes. A number of recent and current projects are studying cesium and strontium in the environment in water, soil, plants sediment and fish to increase understanding of environmental transport mechanisms and biokinetics. The role of organically bound tritium (terrestrial and aquatic) in the environmental cycling of tritium is specifically being investigated to update the science used in predictive models such as the those used by the CNSC and licensees for modelling radionuclide transport.
Of note is a new project commencing in 2017, to develop biokinetic models for cesium and strontium in Great Lakes aquatic food webs.
Part II conclusion
The previous sections have reviewed the current international and national risk management and regulatory frameworks for radionuclides, and provided an outline of current provincial and federal monitoring and research activities. Radionuclides are among the most heavily regulated substances, both internationally and nationally. There is a well-developed internationally recognized protection framework that has effectively kept doses of radiation in workers and the public well below the regulatory limits. Radiation monitoring networks have been established nationally with extensive coordination among provincial and international monitoring networks.
Canada has an independent national nuclear regulatory body (i.e., the CNSC), the mandate of which is to protect the environment and the health and safety of persons. The CNSC has no promotional role for the industry. The CNSC regulatory framework requires licensees to complete site-specific radiation monitoring, while the CNSC itself performs independent monitoring of the environment surrounding regulated nuclear facilities. Strong inter-governmental relationships have been established both federally and provincially to ensure radionuclides and radiation are managed safely.
The comprehensive nature of the current regulatory framework and federal and provincial monitoring and research networks can be evaluated by comparing current practices and structures against Annex 3 commitments for designated Chemicals of Mutual Concern (CMCs). Potential CMC risk-management initiatives with an assessment of the status of such activities within Canada are provided in table 13.
Develop risk-management strategies that may include research, monitoring, surveillance and pollution prevention and control provisions |
|
Develop and implement water-quality standards objectives, criteria and guidelines, subject to domestic law and regulation. |
|
Reduce anthropogenic releases of CMCs and products containing CMCs throughout their entire lifecycles. |
|
Promote the use of safer chemical substances and the use of technologies that reduce or eliminate the uses and releases of CMCs. |
|
Continue progress toward the sound management of CMCs using approaches that are accountable, adaptive and science-based. |
|
Monitor and evaluate the progress and effectiveness of pollution prevention and control measures for CMCs and adapt management approaches as necessary. |
|
Exchange information on monitoring, surveillance, research, technologies and measures for managing CMCs. |
|
|
Coordinate and collaborate with various stakeholders on science priorities, research, surveillance and monitoring activities in the Great Lakes basin ecosystem. |
|
6.0 Conclusions and recommendations
The data on the quantities of radionuclides monitored within the Great Lakes have been summarized and assessed using internationally and nationally recognized environmental and human health risk assessment approaches. The results are compared to science-based benchmarks for protection of the environment and of human health. This assessment, using the large volume of available environmental data and the best science on the effects of radiation on human health and the environment, indicates that there is no evidence to suggest that radionuclides pose an unreasonable risk to environment, health or safety within the Great Lakes basin ecosystem.
Furthermore, radionuclides are among the most heavily regulated substances both internationally and nationally. Canada has an independent national nuclear regulatory body (i.e., the CNSC), the mandate of which is to protect the environment, and the health and safety of persons. The CNSC has no promotional role for the industry. Strong inter-governmental relationships have been established both federally and provincially to ensure radionuclides and radiation are safely managed.
When the comprehensive nature of the current regulatory framework and national monitoring and research network is considered, it is clear that activities are already in place federally which would satisfy all Annex 3 commitments for designated CMCs. Therefore, based on the evaluation summarized in this report, the CNSC has concluded that:
- the health and safety of persons and the environment associated with the Great Lakes are protected
- designating radionuclides as a CMC would not lead to or enable any additional action on the part of the Canadian federal government
As such, it is recommended that Canada does not support the nomination of radionuclides as a candidate CMC for further evaluation.
7.0 References
- Andersson, P., Garnier-Laplace, J., Beresford, N.A., Copplestone, D., Howard, B.J., Howe, P., Oughton, D., and P. Whitehouse. 2009. Protection of the environment from ionising radiation in a regulatory context (protect): proposed numerical benchmark values. Journal of Environmental Radioactivity, Vol. 100, Iss. 12, 1100-1108.
- Baweja, A.S., S.R. Joshi, and A. Demayo. 1987. Radionuclide content of some Canadian surface waters: A report on the national radionuclides monitoring program, 1981-1984. Environment Canada, Inland Waters/Lands Directorate Water Quality Branch, Scientific Series No. 156. 00
- BEIR VII. 2006. Health risks from exposure to low levels of ionizing radiation: BEIR VII phase 2. Committee to assess health risks from Eeposure to low levels of ionizing radiation. United States National Research Council of the National Academies. National Academy Press, Washington.
- Brown, J.E., H. Thørring and A. Hosseini. 2003. The "EPIC" impact assessment framework – a deliverable report for EU Funded Project ICA2-CT-2000-10032. Norwegian Radiation Protection Authority, Østerås, pp. 175.
- Brown, J.E., B. Alfonso, R. Avila, N.A. Beresford, D. Copplestone, G. Pröhl and A. Ulanovsky. 2008. The ERICA tool. Journal of Environmental Radioactivity, 99: 1371-1383.
- Brown, J.E., B. Alfonso, R. Avila, N.A. Beresford, D. Copplestone, and A. Hosseini. 2016. A new version of the ERICA tool to facilitate impact assessments of radioactivity on wild plants and animals. Journal of Radioactivity, 153: 141-148.
- Bruce Power, 2006. Bruce A Refurbishment for Life Extension and Continued Operation Project Environmental Assessment Study Report.
- CELA (Canadian Environmental Law Association). 2016. Radionuclides as a chemical of mutual concern in the Great Lakes basin. Prepared for Canadian Environmental Law Association. Prepared by John Jackson.
- CEU (Council of the European Union). 2013. Council Directive 2013/59/EUROTOM of 5 December 2013 laying down basic safety standards for protection against the dangers arising from exposure to ionising radiation, and repealing Directives 89/618/Euratom, 90/641/Euratom, 96/29/Euratom, 97/43/Euratom and 2003/122/Euratom. Official Journal of the European Union. 17.1.2014.
- Choppin, G.R., J.O. Liljenzin, and J. Rydberg. 2002. Radiochemistry and nuclear chemistry, 3rd edition. Butterworth-Heinemann, Woburn, MA.
- CNSC (Canadian Nuclear Safety Commission). 2010. CMD 10-H19 Submission from CNSC staff on application for Bruce Power for licence to transport 16 steam generators to Sweden.
- CNSC (Canadian Nuclear Safety Commission). 2014. NAC-LWT package design for transport of highly enriched uranyl nitrate liquid. Technical Assessment Report.
- CSA (Canadian Standards Association). 2012. N288.6-12 - Environmental risk assessments at class I nuclear facilities and uranium mines and mills.
- CSA (Canadian Standards Association). 2014a. N288.1-14 - Guidelines for calculating derived release limits for radioactive material in airborne and liquid effluents for normal operation of nuclear facilities.
- CSA (Canadian Standards Association). 2014b. N292.0-14 - General principles for the management of radioactive waste and irradiated fuel.
- DOE (Department of Energy). 2002. A graded approach for evaluating radiation doses to aquatic and terrestrial biota. U.S. Department of Energy, Washington, D.C., U.S. Technical Standard DOE-STD-1153-2002.
- DOE (Department of Energy) 2004. RESRAD-BIOTA: A tool for implementing a graded approach to biota dose evaluation. U.S. Department of Energy, Washington, D.C., ISCORS Technical Report 2004-02, DOE/EH-0676.
- EC and HC (Environment Canada and Health Canada) 2004. Releases of radionuclides for nuclear facilities (impact on non-human biota). Priority Substance List Assessment Report prepared for the Canadian Environmental Protection Act - May 2003. TD193.N27 2003, ISBN 0-662-35410-9.
- Garnier-Laplace, J. and R. Gilbin (Eds). 2006. Derivation of predicted-no-effects-dose-rate values for ecosystems (and their sub-organizational levels) exposed to radioactive substances. Report D5 to the ERICA project (EC Contract number F16R-CT-2003-508847). Swedish Radiation Protection Authority, 88 pp.
- Garnier-Laplace, J., D. Copplestone, R. Gilbin, F. Alonzo, P. Ciffroy, M. Gilek, A. Agüero, M. Björk, D.H. Oughton, A. Jaworska, C.M. Larsson, and J. Hingston. 2008. Issues and practices in the use of effects data from FREDERICA in the ERICA Integrated Approach. Journal of Environmental Radioactivity, 99: 1474-1483.
- Grasty, R.L. and J.R LaMarre. 2004. The annual effective dose from natural sources of ionising radiation in Canada. Radiation Protection Dosimetry, vol 108(3), 215-226.
- HC (Health Canada) 2001. Canadian guidelines for the restriction of radioactively contaminated food and water following a nuclear emergency guidelines and rationale. Minister of Public Works and Government Services Canada, Cat. H46-2/01-254E.
- HC (Health Canada) 2003. Canadian guidelines for intervention during a nuclear emergency. Published by authority of the Minister of Health. Her Majesty the Queen in Right of Canada, Cat. H46-2/03-326E.
- HC (Health Canada) 2004. Federal contaminated sites risk assessment in Canada – Part I: Guidance on human health preliminary quantitative risk assessment (PQRA).
- HC (Health Canada) 2009. Guidelines for canadian drinking water quality: Guideline technical document - radiological parameters. Radiation Protection Bureau, Healthy Environments and Consumer Safety Branch, Health Canada, Ottawa, Ontario. (Catalogue No. H128-1/10-614E-PDF).
- HC (Health Canada). 2011. Canadian guidelines for the management of naturally occurring radioactive materials (NORM). Prepared by the Canadian NORM working group of the Federal Provincial Territorial Radiation Protection Committee. Cat.: H129-34/2013E-PDF.
- HC (Health Canada). 2015. Special environmental radiation in canada report on fukushima accident contaminants technical report surveillance of fukushima emissions in Canada: March 2011 to June 2011. Health Canada Radiation Protection Branch. ISBN 978-0-660-02800-2.
- HPS (Health Physics Society). 2016. Radiation risk in perspective – position statement of the Health Physics Society. Health Physics Society. Position Statement 110-3. Adopted 1996. Revised 2010, 2013.
- IAEA (International Atomic Energy Association). 1998. Clearance of materials resulting from the use of radionuclides in medicine, industry and research. IAEA technical document, IAEA-TECDOC-1000, International Atomic Energy Association, Austria ISSN-1011-4289
- IAEA (International Atomic Energy Association). 2003. Radiation protection and the management of radioactive waste in the oil and gas industry. Safety Report Series 34. International Atomic Energy Association, Austria STI/PUB/11171.
- IAEA (International Atomic Energy Association). 2013. Radiation protection and management of norm residues in the phosphate industry. Safety Report Series 78. International Atomic Energy Association, Austria STI/PUB/1582.
- IAEA (International Atomic Energy Association). 2014a Handbook of parameter values for the prediction of radionuclide transfer to wildlife. Technical Report Series No. 479. International Atomic Energy Association, Austria STI/DOC/010/479.
- IAEA (International Atomic Energy Association) 2014b. Radiation protection and safety of radiation sources: international basic safety standards, general safety requirements Part 3 No. GSR Part 3. International Atomic Energy Association, Austria STI/PUB/1578.
- IAOGP (International Association of Oil and Gas Producers). 2008. Guidelines for the management of naturally occurring radioactive material (NORM) in the oil and gas industry, report No. 412.
- IJC (International Joint Commission). 1983. 1983 Report on Great Lakes water quality -- appendix on radioactivity. Great Lakes Water Quality Board, International Joint Commission, Windsor. In, Joshi, S.R. 1991. Radioactivity in the Great Lakes. The Science of the Total Environment Vol. 100, 61-104.
- Joshi, S.R. 1991. Radioactivity in the Great Lakes. The Science of the Total Environment Vol. 100, 61-104.
- King-Sharp, K.J. 2009. Tritium in the Great Lakes 2008: Re-evaluation of the Great Lakes tritium model. Technical Note: TN-08-3039. CANDU Owners Group Inc.
- Klukas, M.H. 1999. Tritium in the Great Lakes: concentration – time model. Atomic Energy of Canada report, RC-2247.
- Larsso, C.M. 2016. Overview of ICRP Committee 5: protection of the environment. Annals of the ICRP, Vol 45(Iss. 1) supplemental.
- NPRB (National Radiological Protection Board). 1998. Revised generalised derived limits for radioisotopes of strontium, ruthenium, iodine, caesium, plutonium, americium and curium. NRPB Volume 9 number 1. ISBN 0 85951 413 7.
- NY (New York) DEC (Department of Environmental Conservation). 2014. Assessment of the risks to fish and wildlife from exposure to ionizing radiation. New York State Department of the Environmental Conservation, Division of Fish, Wildlife and Marine Resources.
- Ontario Hydro. 1987. Annual summary and assessment of environmental radiological data for 1986. Safety Services Department, Rep. SSD-AR-86-1, Toronto. In, Joshi, S.R. 1991. Radioactivity in the Great Lakes. The Science of the Total Environment Vol. 100, 61-104.OPG (Ontario Power Generation). 2007. Refurbishment and Continued Operation of Pickering B Nuclear Generating Station Environmental Assessment.
- U.K. Environment Agency. 2010. Radioactive substances regulation – Environmental principles. Regulatory guidance series, No RSR 1. Government of the United Kingdom Environment Agency. https://www.gov.uk/government/publications/radioactive-substances-regulation-environmental-principles
- UNEP (United Nations Environment Programme). 2016. Radiation: effects and sources, United Nations Environment Programme, Austria ISBN: 978-92-807-3517-8.
- UNSCEAR (United Nations Scientific Committee on the Effects of Atomic Radiation). 2010. Sources and effects of ionizing radiation UNSCEAR 2008 report to the general assembly with scientific annexes. Volume I, Annex B. Exposures of the public and workers from various sources of radiation. United Nations New York Publication ISBN: 978-1-142274-0.
- UNSCEAR (United Nations Scientific Committee on the Effects of Atomic Radiation). 2015. Sources and effects of ionizing radiation, UNSCEAR 2012 report to the general assembly with scientific annexes A and B. Annex A. Attributing health effects to ionizing radiation exposure and inferring risks. United Nations New York Publication ISBN: 978-92-1-142307-5.
- UNSCEAR (United Nations Scientific Committee on the Effects of Atomic Radiation). 2017. Sources and effects of ionizing radiation, UNSCEAR 2016 report to the general assembly with scientific annexes A, B, C and D. Annex B: Radiation exposures from electricity generation.
- WHO (World Health Organization). 2004. Guidelines for drinking-water quality 3rd Edition Volume 1 recommendations. World Health Organization Geneva. ISBN 92 4 154638 7.nited Nations New York ISBN: 978-92-1-142316-7.
- Yim, M-S and F. Caron. 2006. Life cycle and management of carbon-14 from nuclear power generation. Progress in Nuclear Energy, Vol. 48: 2-36.
ACRONYMS
- ALARA
- as low as reasonably achievable
- APM
- Adaptive Phase Management
- BATEA
- best available technology economically achievable
- BEIR
- Biological effects of ionizing radiation
- Bq
- bequerel
- BRR
- Blind River Refinery
- CANDU
- CANada Deuterium Uranium
- CELA
- Canadian Environmental Law Association
- CEPA
- Canadian Environmental Protection Act
- CMC
- Chemical of Mutual Concern
- CNSC
- Canadian Nuclear Safety Commission
- COMET
- Coordination and implementation of a pan-Europe instrument for radioecology
- CRMN
- Canadian Radiological Monitoring Network
- CSA
- Canadian Standards Association
- DGR
- Deep Geologic Repository
- EA
- environmental assessment
- ECCC
- Environment and Climate Change Canada
- ERA
- environmental risk assessment
- ERAP
- emergency response assistance plan
- ERICA
- Environmental Risk from Ionising Contaminants: Assessment and Management
- FASSETT
- Framework for the Assessment of Environmental Impact
- FNSTP
- Federal Nuclear Science and Technology Program
- FPS
- Fixed Point Surveillance
- FPTRPC
- Federal Provincial and Territorial Radiation Protection Committee
- GLWQA
- Great Lakes Water Quality Agreement
- Gy
- gray
- HC
- Health Canada
- HLRW
- high-level radioactive waste
- IAEA
- International Atomic Energy Agency
- ICRP
- International Commission of Radiation Protection
- IEMP
- Independent Environmental Monitoring Program
- ILRW
- intermediated-level radioactive waste
- IRRS
- Integrated Regulatory Review Service
- ISRP
- International System of Radiological Protection
- LLRW
- low-level radioactive waste
- LNT
- linear-no-threshold
- MDL
- method detection limit
- NCRP
- National Council on Radiation Protection and Measurements
- NORM
- naturally occurring radioactive material
- NPP
- nuclear power plant
- NSCA
- Nuclear Safety and Control Act
- NWMO
- Nuclear Waste Management Organization
- PHAI
- Port Hope Area Initiative
- PHCF
- Port Hope Conversion Facility
- PROTECT
- Protection of the environment from ionising radiation in a regulatory context
- PTNSR
- Packaging and Transport of Nuclear Substances Regulations
- ROR
- regulatory oversight report
- STAR
- Strategy for Allied Radioecology
- Sv
- sievert
- TDG
- Transportation of Dangerous Goods
- UNSCEAR
- United Nations Scientific Commission on the Effects of Atomic Radiation
- WMF
- Waste Management Facility
Appendix A: CNSC radionuclide single media screening criterion – fish consumption
The CNSC has based its development of screening levels on an annual effective dose to a member of the public of 0.1 mSv/year. This screening dose is one tenth of the annual public dose limit, and more than an order of magnitude lower than the average exposure to Canadians from natural background radiation of 1.8 mSv per year (Grasty and Lamare 2004). This screening dose was chosen to account for potential exposure to other pathways and media. This approach is consistent with that used to develop Health Canada's Guidelines for Canadian Drinking Water Quality (HC 2009), the World Health Organization's Guidelines for Drinking-water Quality (WHO 2004), and the United Kingdom's generalized derived limits (NPRB 1998).
Exposure modelling calculations are based on the environmental transfer and pathways exposure equations in CSA N288.1 (CSA 2014a), the approved Canadian standard for the calculation of public dose from environmental releases. Calculations were made for the three age classes identified in table A1 to account for differences in dietary consumption rates and lifestyle habits. For each radionuclide in each type of environmental media, the most conservative screening level is selected.
Designation | Age range (years) | Nominal age for characteristics and dose coefficients |
---|---|---|
Infant | 0–5 | 1 year |
Child | 6–15 | 10 years |
Adult | 16–70 | Adult |
Default model parameters for dose coefficients and ingestion rates for the age classes were selected from CSA N288.1-14. For example, ingestion rates for each age class were represented by the 95th percentile of their distribution (i.e., adult: 28.1, child: 18.5, infant: 6.4 g fresh weight (fw)/day).
Screening levels in Bq (kg fw)-1 for fish ingestion (SLfish) were calculated using equation 1.
where, P(i)69 is the fish ingestion dose coefficient (Sv yr-1 Bq-1 kg fw) calculated using equation 2
where, Ifish is daily ingestion rate in units of g fw day-1 for the type of food; ƒlocal is the unitless local fraction of fish consumed; DCFƒ is the ICRP dose coefficient for intake by ingestion for members of the public (Sv Bq-1); and dyear is the number of days in a year (i.e., 365).
Example calculations of the generic screening level for fish consumption containing tritium are provided below.
In this case, the adult screening level is selected. Examples of calculated screening levels for fish ingestion are provided in table A2.
Radionuclide | Fish screening level (Bq/kg fresh weight) |
---|---|
Cesium-134 | 710 |
Cesium-137 | 1,040 |
Carbon-14 | 16,800 |
Cobalt-60 | 1,350 |
Potassium-40 | 1,020 |
Tritium (HTO) | 488,000 |
Appendix B: Human health risk assessment: public dose from Great Lakes exposure pathways
The methodology to ascertain doses to persons as a result of radionuclides in the Great Lakes is consistent with the method detailed in CSA N288.1-14 (CSA 2014a).
Representative persons
An individual with characteristics that reflect those of the group that receives the highest doses from a particular source is referred to as a representative person (CSA 2014a). Eight representative persons were included in this assessment.
Lake Ontario residents include:
- non-Indigenous persons residing near nuclear power plants
- non-Indigenous persons residing near facilities discharging radionuclides of the uranium-238 series
- Indigenous persons residing near nuclear power plants
- Indigenous persons residing near facilities discharging radionuclides of the uranium-238 series
Lake Huron residents include:
- non-Indigenous persons residing near nuclear power plants
- non-Indigenous persons residing near facilities discharging radionuclides of the uranium-238 series
- Indigenous persons residing near nuclear power plants
- Indigenous persons residing near facilities discharging radionuclides of the uranium-238 series
Data used in the assessment
Environmental monitoring data from 2006 to 2015 were used to generate summary statistics for the 10-year assessment period. The specific data used as input variables are shown in table B1.
Facility | Sample type | Parameter |
---|---|---|
Darlington Nuclear Generating Station | Fish | Tritium (HTO) |
Tritium (OBT) | ||
Carbon-14 | ||
Water (lake) | Tritium (HTO) | |
Cobalt-60 | ||
Cesium-137 | ||
Water (water supply plant) | Tritium (HTO) | |
Total beta (assumed to be strontium-90) | ||
Sediment | Cesium-137 | |
Pickering Nuclear Generating Station | Fish | Tritium (HTO) |
Tritium (OBT) | ||
Carbon-14 | ||
Water (lake) | Tritium (HTO) | |
Cobalt-60 | ||
Cesium-137 | ||
Water (water supply plant) | Tritium (HTO) | |
Total beta (assumed to be strontium-90) | ||
Sediment | Cesium-137 | |
Bruce A and B nuclear generating stations | Fish | Tritium (HTO) |
Water (lake) | Tritium (HTO) | |
Total beta (assumed to be cobalt-60) | ||
Water (water supply plant) | Tritium (HTO) | |
Total beta (assumed to be strontium-90) | ||
Sediment | Cesium-137 | |
Port Hope Conversion Facility | Water (lake) | Total uranium |
Water (water supply plant) | Total uranium | |
Port Granby Project | Water (lake) | Total uranium |
Polonium-210 | ||
Radium-226 | ||
Elliot Lake | Water (lake) | Total uranium |
Radium-226 | ||
Water (water supply plant) | Total uranium | |
Radium-226 | ||
Sediment | Total uranium | |
Blind River Refinery | Water (lake) | Total uranium |
Water (water supply plant) | Total uranium |
Exposure pathways
The following exposure pathways associated with radionuclides in Great Lakes water or aquatic environment were included:
- ingestion of drinking water
- immersion in lake water (e.g., from swimming)
- external exposure to sediment
- ingestion of fish
Human exposure factors
The ingestion rates used in the dose assessment are shown in table B2, with dose coefficients for ingestion and external exposure from sediment taken from CSA N288.1-14 (CSA 2014).
Parameter | Value |
---|---|
Drinking water ingestion rates | |
Adult | 2.96 L/d |
Child | 1.32 L/d |
Infant | 0.837 L/d |
Indigenous adult | 2.96 L/d |
Indigenous child | 1.32 L/d |
Indigenous infant | 0.837 L/d |
Fish ingestion rates | |
Adult | 28.1 g (fw)/d |
Child | 18.5 g (fw)/d |
Infant | 6.4 g (fw)/d |
Indigenous adult | 220 g (fw)/d |
Indigenous child | 170 g (fw)/d |
Indigenous infant | 95 g (fw)/d |
Consideration of background data and averaging
For each facility, sample type and parameter, exposure and reference data were extracted from the environmental monitoring data associated with CNSC regulated facilities within the Great Lakes. The maximum monitoring result for each year was carried forward in the assessment. For each year, the average value of reference data was selected, and used for background correction.
Calculation method
The net concentrations calculated as described above were then used as input into the equations for each relevant pathway, as set out in CSA N288.1-14 (CSA 2014a). Where radionuclide concentration data in fish were not available, namely those radionuclides associated with the uranium-238 series, these were modelled from lake water data. The modelling approach is described in section 7.7 of CSA N288.1-14 (CSA 2014a), by applying the bioaccumulation factors from CSA N288.1-14 table A25a [Default values of P26 (BAF), transfer from water to fish muscle for freshwater fish (L/kg ƒw)].
The annual dose thus calculated, by facility and by year, for each parameter was summed to yield a year-by-year dose for each exposure pathway. The annual doses by pathway were summed to yield the total dose, by facility, by year. The year with the maximum annual dose was retained as the representative dose for each representative person. Annual doses were calculated for infants, children and adults. The maximum annual dose to residents near nuclear power plants and residents near facilities releasing uranium series radionuclides were reported for both of these types of facilities.
Appendix C: Radiological ecological risk assessment
ERICA Assessment Tool (Version 1.2) analyses – Benthic and Pelagic Fish
Receptor | Tritium | Gross beta |
---|---|---|
Benthic fish: exposure | ||
Bruce | 35.6 | 0.09 |
Pickering | 11.6 | 0.11 |
Darlington | 9.17 | 0.13 |
Pelagic fish: exposure | ||
Bruce | 35.6 | 0.09 |
Pickering | 11.6 | 0.11 |
Darlington | 9.17 | 0.13 |
Benthic fish: reference | ||
Bruce | 3.10 | 0.08 |
Pickering | 3.10 | 0.08 |
Darlington | 3.10 | 0.08 |
Pelagic fish: reference | ||
Bruce | 3.10 | 0.08 |
Pickering | 3.10 | 0.08 |
Darlington | 3.10 | 0.08 |
Comments
- Analyses completed using ERICA Tier II defaults with conservative assumptions resulting in maximum potential dose.
- Gross beta is represented by strontium-90 used in this dose rate assessment using the ERICA Assessment Tool (Version 1.2).
ERICA Assessment Tool (Version 1.2) analyses – Benthic and Pelagic Fish
Receptor | Cesium-137 | Cesium-134 | Cobalt-60 | Carbon-14 |
---|---|---|---|---|
Benthic fish: exposure | ||||
Bruce | 2.08 | 0.26 | 0.38 | 19.22 |
Pickering | 2.44 | 0.44 | 0.51 | 19.22 |
Darlington | 0.48 | 0.32 | 0.34 | 12.48 |
Pelagic fish: exposure | ||||
Bruce | 2.08 | 0.26 | 0.38 | 19.22 |
Pickering | 2.44 | 0.44 | 0.51 | 19.22 |
Darlington | 0.48 | 0.32 | 0.34 | 12.48 |
Benthic fish: reference | ||||
Bruce | 0.66 | 0.30 | 0.31 | 14.4 |
Pickering | 0.66 | 0.30 | 0.31 | 14.4 |
Darlington | 0.66 | 0.30 | 0.31 | 14.4 |
Pelagic fish: reference | ||||
Bruce | 0.66 | 0.30 | 0.31 | 14.4 |
Pickering | 0.66 | 0.30 | 0.31 | 14.4 |
Darlington | 0.66 | 0.30 | 0.31 | 14.4 |
Comments
- Analyses completed using ERICA Tier II defaults with conservative assumptions resulting in maximum potential dose.
- Values for carbon-14 were reported as Bq/kg C, therefore, these values were corrected for organic carbon content in the sediment. The highest mean total organic carbon content of sediment (i.e., 9.6%) was used in this correction, based on mean measured values (1.8% to 9.6%) reported (OPG, 2014), resulting in a conservative estimate of carbon-14 in the sediments.
- Carbon-14 values for the Bruce nuclear generating stations were not available, therefore the highest available value measured at the Pickering Nuclear Generating Station was used for modelling dose to aquatic biota, based on general similarities between the Bruce and Pickering NPPs (e.g. number of reactors, years of operation and CANDU design).
- Sediment carbon-14 values used in this assessment are likely an overestimate because they include carbon-14 produced naturally in the upper atmosphere, by the irradiation of nitrogen-14 by neutrons of cosmic ray origin, which imparts natural radioactivity to carbon present in the atmosphere and the biosphere due to mixing with stable isotopes of carbon (Yim and Caron 2006). Also, past atmospheric testing of nuclear weapons has contributed substantial carbon-14 to the carbon reservoir in the environment (Choppin et al. 2002).
RESRAD-BIOTA (Version 1.5) analyses – Aquatic Animal and Riparian Animal
Receptor | Tritium | Gross Beta |
---|---|---|
Aquatic animal: exposure | ||
Bruce | 35.6 | 0.09 |
Pickering | 11.6 | 0.11 |
Darlington | 9.17 | 0.13 |
Riparian animal: exposure | ||
Bruce | 35.6 | 0.09 |
Pickering | 11.6 | 0.11 |
Darlington | 9.17 | 0.13 |
Aquatic animal: reference | ||
Bruce | 3.10 | 0.08 |
Pickering | 3.10 | 0.08 |
Darlington | 3.10 | 0.08 |
Riparian animal: reference | ||
Bruce | 3.10 | 0.08 |
Pickering | 3.10 | 0.08 |
Darlington | 3.10 | 0.08 |
Comments
- Analyses completed using RESRAD-BIOTA Tier II defaults with conservative assumptions resulting in maximum potential dose.
- Gross beta is represented by Sr-90 used in this dose rate modelling using RESRAD-BIOTA.
RESRAD-BIOTA (Version 1.5) analyses – Aquatic Animal and Riparian Animal
Receptor | Cesium-137 | Cesium-134 | Cobalt-60 | Carbon-14 |
---|---|---|---|---|
Aquatic animal exposure | ||||
Bruce | 2.08 | 0.26 | 0.38 | 19.22 |
Pickering | 2.44 | 0.44 | 0.51 | 19.22 |
Darlington | 0.48 | 0.32 | 0.34 | 12.48 |
Riparian animal exposure | ||||
Bruce | 2.08 | 0.26 | 0.38 | 19.22 |
Pickering | 2.44 | 0.44 | 0.51 | 19.22 |
Darlington | 0.48 | 0.32 | 0.34 | 12.48 |
Aquatic animal reference | ||||
Bruce | 0.66 | 0.30 | 0.31 | 14.4 |
Pickering | 0.66 | 0.30 | 0.31 | 14.4 |
Darlington | 0.66 | 0.30 | 0.31 | 14.4 |
Riparian animal reference | ||||
Bruce | 0.66 | 0.30 | 0.31 | 14.4 |
Pickering | 0.66 | 0.30 | 0.31 | 14.4 |
Darlington | 0.66 | 0.30 | 0.31 | 14.4 |
Comments
- Analyses completed using RESRAD-BIOTA Tier II defaults with conservative assumptions resulting in maximum potential dose.
- Values for carbon-14 were reported as Bq/kg C, therefore, these values were corrected for organic carbon content in the sediment. The highest mean total organic carbon content of sediment (i.e., 9.6%) was used in this correction, based on mean measured values (1.8% to 9.6%) reported (OPG, 2014), resulting in a conservative estimate of carbon-14 in the sediments.
- Carbon-14 values for the Bruce nuclear generating stations were not available, therefore the highest available value measured at the Pickering Nuclear Generating Station was used for modelling dose to aquatic biota, based on general similarities between Bruce and Pickering NPPs (e.g., number of reactors, years of operation and CANDU design).
- Sediment carbon-14 values used in this assessment are likely an overestimate because they include carbon-14 produced naturally in the upper atmosphere, by the irradiation of nitrogen-14 by neutrons of cosmic ray origin, which imparts natural radioactivity to carbon present in the atmosphere and the biosphere due to mixing with stable isotopes of carbon (Yim and Caron 2006). Also, past atmospheric testing of nuclear weapons has contributed substantial carbon-14 to the carbon reservoir in the environment (Choppin et al. 2002).
Related links
Footnotes
- Footnote 1
-
Sampling ceased in 1999 as U and Ra-226 were below federal drinking water guidelines.
- Footnote 2
-
Sampling ceased in 2004 as U and Ra-226 were below federal drinking water guidelines.
- Footnote 3
-
Target species are lake whitefish and white sucker. Back-up species are brown bullhead and round whitefish.
- Footnote 4
-
Uranium mining in Canada is considered to be part of the nuclear fuel cycle and is thus regulated by the CNSC.
- Footnote 5
-
A synopsis is available at https://www.ec.gc.ca/ese-ees/default.asp?lang=En&n=2A379917-1#a1
- Footnote 6
-
http://nuclearsafety.gc.ca/eng/resources/publications/reports/uranium/index.
- Footnote 7
-
RESRAD-BIOTA model applies the 10 CFR 834 Subpart F, 10 mGy/day limit as the screening benchmark. This equates to 416 µGy/h and as such is identical to the aquatic screening benchmarks proposed by UNSCEAR and the IAEA (i.e., 400 µGy/h).
Page details
- Date modified: